Figure 6.1 Hydrophilic colloid
Hydrophilic groups attached to a colloidal particle can help particle stability by adding more repulsion between particles via electrostatic forces.
Lyophobic colloids, in contrast (to lyophilic colloids), are actually stabilized via repulsion between dispersed particles and the dispersion medium (Figure 6.2).
Lyophobic (hydrophobic if an aqueous dispersion medium) colloids are large collections of ions and are thermodynamically unstable systems, possessing little stabilizing interaction between the dispersed phase and the dispersion medium due to low adhesional forces between the two phases. Lyophobic colloids do not form spontaneously, but rather require special techniques to create them. Stability for lyophobic colloids is achieved through ensuring balanced repulsion both within the dispersed phase and between the dispersed phase and dispersion medium. Hydrophobic colloids are stabilized only through electrical repulsion among particles – and between particles and the aqueous dispersion medium. When part of a more complex delivery system, hydrophobic colloids do not alter the system viscosity with increasing concentration. Examples of hydrophobic colloids are silver iodide and gold sols.
Figure 6.2 Adsorbed ions acting to stabilize hydrophobic colloidal particles
Ions can sometimes be adsorbed onto the dispersed particle surface, providing repulsion (VR involves mainly electrostatic repulsion forces, discussed later in this chapter) among dispersed particles and imparting a degree of attraction between the hydrophobic dispersed phase and the dispersion medium. Both repulsive and attractive forces are necessary to provide stability to this type of colloid.
Association (or amphiphilic, meaning solvent and solute ‘loving’) colloids are thermodynamically stable systems in which the dispersed phase consists of aggregates of amphiphilic molecules or ions (micelles) that have strong interactions between the dispersed phase and dispersion medium, and so can act as surfactants (Figure 6.3). These colloids possess hydrophilic and hydrophobic regions within the same molecule, which allow the favorable interactions with the dispersion medium and the dispersed phase. Aqueous-based association colloids are stabilized via both hydration of the dispersed particles and by hydrophobic interactions that create micelles. At low dispersed phase concentrations, the molecules exist in true solution (Figures 6.4 and 6.5). At higher concentrations, they spontaneously aggregate above the critical micelle concentration (CMC) to form micelles.
When part of a more complex delivery system, hydrophilic colloids exhibit little change in viscosity, though some increase the delivery system viscosity when at high concentrations. Examples of aqueous association colloids are those created with Tween 80 and bile salts.
The common characteristic of all types of colloids is the interaction between the dispersed phase and the dispersion medium. When controlled, any type can remain dispersed. Controlling colloidal dispersions depends on maintaining optimum balances of attractive and repulsive forces between phases. Therefore, we will take a closer look at these forces. Lyophilic and lyophobic colloids will be discussed first, followed by association colloids.
Figure 6.3 Cross-section of a micelle
In this illustration, the long portions are hydrophobic, while the small balls are hydrophilic. When dispersed at lower concentrations (below the critical micelle concentration), these amphiphiles can act as surfactants, stabilizing colloidal dispersions.
Figure 6.4 Increasing amphiphile concentration creates micelles
In (A), the concentration of amphiphile is below the critical micelle concentration (CMC). As amphiphile concentration increases, (B) illustrates a concentration of amphiphile still below the CMC, and (C) at a point where the dispersion phase surface is saturated. After surface saturation (above the CMC), amphiphiles form micelles in order to minimize potential energy (D).
Figure 6.5 Amphiphile (surfactant) concentration and critical micelle concentration (CMC)
As the surfactant concentration increases, surface tension decreases until the CMC is reached. After the CMC is reached, no further decrease in surface tension occurs because the interface is saturated.
Stabilizing forces for colloids
I. Lyophilic and lyophobic colloids
As mentioned, the dispersed phase and dispersion medium of lyophobic colloids do not interact well with regard to stabilizing the system. The result is that not only can these colloids be difficult to make, but they also are sensitive to surrounding conditions, such as changing ionic strength or pH. The stability of any colloidal system requires keeping the dispersed phase dispersed (i.e., not coagulated). Therefore, the dispersed phase should not be overly attracted to either the dispersion medium (adhesion) or the other dispersed particles (cohesion). The condition of being dispersed creates a thermodynamic instability: the dispersed particles possess potential energy (PE) that is not difficult to lose. By aggregating with other particles this PE is lost, but then so is our drug delivery system. Lyophobic colloids have a propensity to coagulate in order to gain thermodynamic stability, and this is not what we wish to occur. In order for disperse drug delivery systems to be successful, they must remain in a dispersed condition. Dispersed particles of hydrophobic colloids (the most common useful type of lyophobic colloids) have a natural lack of affinity for water, and so have a drive to separate from the water. They can be prepared in water only if stabilized in some way – either by adsorption of ions onto their surfaces or by providing hydrophilic groups, that will interact positively with water, to their surfaces. This allows a modicum of affinity for the dispersion medium, offsetting the natural attraction between particles, hopefully adequate to keep the dispersed particles apart. Hydrophobic colloids do not spontaneously disperse in aqueous media (as hydrophilic colloids do). So, providing some measure of affinity for water, as well as ensuring colloidal particles repel each other, provides dispersion and stability.
Disperse systems can be viewed as balances of potential energy. Keep in mind this is applicable to all disperse systems, including colloids, suspensions and emulsions. For now, the discussion is focused on colloids. What are the attractive and repulsive forces at work? Attractive forces include van der Waals interactions and the electrical attractions between disparately charged particles or portions of particles. Repulsive forces include electrical repulsion between particles or portions of particles for any colloid type. Also, steric hindrance is a repulsive force. In some instances, steric effects due to the actions of components intentionally added to the colloidal preparation can add resistance to particle aggregation. For hydrophilic and association colloids, hydration (stabilization via proximal water molecules) of particles and solvation actions (even though a solution is not made) upon particles are contributing forces. A simple mathematical representation of the interacting forces on colloidal particles can be represented using the following general equation:
where
VT = the total (net) force
VA = the total of attractive forces (van der Waals, adsorbed ions)
VR = the electrostatic repulsive forces
VS = steric repulsion.
VA is traditionally a negative value, indicating attraction. VR and VS are traditionally positive values, indicating repulsion.
To illustrate the actions of those forces applicable to hydrophobic colloids, Figures 6.6 to 6.9 are used. The forces result in PEs of attraction and repulsion, along with a net PE along the distance from a charged particle’s surface. The net force between particles and dispersion medium, VT, is the resulting sum of forces that attract the particles to the dispersion medium, attract particles to one another, and those that repel particles from the dispersion medium and those forcing repulsion between particles (Figure 6.6). For a stable dispersion, the goal is to achieve a VT as close to zero as possible (i.e., no net force). A closer look at the interparticulate (cohesive) forces of the dispersed particles will be taken.
Keeping the dispersed phase particles apart is a major requirement for disperse systems. The distance at which particles are kept is the heart of this requirement. Two charged particles (any dispersion type, but for now colloidal particles) can be viewed as possessing both attractive and repulsive forces between them, as illustrated in Figures 6.7 to 6.9. The steric forces (VS) act over a fairly definite range and remain relatively constant. The electrical forces (VA, VR), however, vary in their net action on two charged particles, depending on the distance between the charged particles.
Figure 6.7 Steric forces acting among particles
This is part of VS = steric repulsive forces. Attaching a lyophobic substance to colloidal particles can physically inhibit close approach of other dispersed phase particles (steric hindrance).
Figure 6.8 van der Waals attractive forces acting among particles
This is part of VA = the total of van der Waals attractive forces. Note that pair interactions are additive. The additive effect of pair interactions is a reason why (especially) lyophobic colloids tend to coagulate. The result of VA (van der Waals attraction illustrated) can either be stabilizing or destabilizing, depending on the strength of interactions. If VA is too strong, coagulation of dispersed particles can result.
Figure 6.9 Electrostatic repulsive forces between lyophobic colloidal particles
This is part of VR = electrostatic repulsive forces. Represented here are particles with a surface charge, with ions surrounding each particle, in a ‘diffuse area.’ If colloidal particles naturally are charged, this can add stability to the particles by preventing coagulation. Obviously, changing the medium pH, or adding electrolytes, can affect VR. Represented here are positively charged colloidal particles surrounded by a layer of similarly and oppositely charged ions. The ‘core’ charge of the colloidal particles acts through this ‘diffuse layer,’ causing particles to repel one another. As will be described, two positively charged particles (colloids for the present discussion) will be most stable at a specific distance from each other. At this distance, particles are attracted by the ‘diffuse areas’ around their counterparts, while balancing attractions with repulsion between the positive charges of the actual particles.
Attractive forces between dispersed particles (VA) act over greater distances than do repulsive forces (VR).
The electrical repulsive and attractive forces (VA and VR) that contribute to the net force (VT) acting between two charged particles are unequal in the ranges over which they act. It is this disparity of range that presents the major complexity for disperse systems: what is the ‘best’ distance to keep particles, and can the particles actually be kept at that distance? Attractive forces act over longer ranges than do repulsive forces: the PE of attractive forces declines with inverse proportion to r6, whereas repulsive forces decline with inverse proportion to r9, where r is distance between molecules. The disparate PEs (normally in units of millivolts, mV) are graphed in Figure 6.10, along with the net PE.
Figure 6.10 Ranges of interparticulate attractive and repulsive forces acting on charged particles
When two like-charged particles are considered, it is found that forces of attraction (VA) act at distances longer than do repulsive forces (VR, VS). The net potential energy (PE; VT) is repulsive at very close range, while attractive forces dominate at intermediate distances, becoming equal (both declining to zero) at larger ranges. These variations in dominant forces are reflected in the net potential energy. Where the net PE is minimized are distances at which charged particles are most likely to reside, while still remaining, to some degree, separated. These PE minima are also referred to as PE wells, and are distances at which dispersed particles will be most stable. Not shown is a second net PE minimum, further away, which will be discussed later in this chapter.
By convention, attractive PE is assigned a negative value, while repulsive PE is assigned positive values. By plotting 1/r9, a typical repulsion curve representing the PE of repulsion (related to VR) is created, and is labeled ‘repulsive PE.’ The plot of –1/r6 represents the attractive PE at varying distances between the two charged particles, representing the attractive PE, VA. Finally, the plot of difference between the attractive and repulsive functions results in the net PE values, related to VT.
There are specific distances where net potential minima occur, where particles are most likely to reside, and result in the most stable dispersed systems.
Figure 6.10 is important because it reveals that, as a result of the differing ranges of forces, there is a certain separation distance that offers the most overall electrical stability to charged particles, based on the net PE at these separation distances. This is a PE well where the net PE is most disparately influenced by attractive forces. For disperse systems there are usually two PE minima, as shown in the embellished Figure 6.11. One minimum (the outer) occurs where there is optimal separation distance between two dispersed particles. This distance is beneficial to stability of the disperse system and is the distance that is preferred because, if a disperse system does decompose but the particles can be held at the outer minimum, the disperse system is energetically much easier to redisperse. The second (inner) PE minimum occurs at a distance between particles that may be detrimental. For colloidal particles, the inner minimum represents such a close approach between two particles that interpenetration of (collision between) the particles is likely. Interpenetration or collision means ‘aggregation’ or ‘coagulation’ of the colloid is likely. Recovering from this closer, larger magnitude PE well is difficult, or perhaps even impractical, due to the amount of energy that would be required to overcome the PE barrier. What this illustrates is that, if a dispersed phase is not stabilized at the outer distance where the energy minimum occurs, the resulting coagulation may be irreversible and the dosage form ruined.
Because there is often a propensity toward interpenetration between dispersed phase components, especially in systems of lyophobic colloids, care must be exercised when considering altering conditions of the disperse system. Any part of the delivery system that can inhibit electrical attraction between the dispersed phase and the dispersion medium, or the repulsion between dispersed particles of a hydrophobic colloidal (i.e., added electrolytes), can destabilize the colloid. Other potentially disruptive events that can occur include temperature and pH changes. As hydrophobic particles are repelled by water, one stabilizing effect imparted by water is a repulsive force toward the dispersed particles. However, provided enough impetus in the form of added electrolytes, acids, bases or temperature change, the protection of the dispersed phase can be compromised, and coagulation can yet occur. In the end, the stability of colloids – and other disperse systems – is dependent upon keeping the particles of the dispersed phase an optimum distance apart at the second (outermost) net PE minimum, which is visualized in Figure 6.12.
Figure 6.11 Outer net potential energy (PE) well
This figure illustrates that 1) repulsive forces dominate when two charged particles are extremely close – to a point that the energy required to be expended to move the particles closer together makes it very unlikely to occur; 2) the attractive potential is predominant at moderate, but still relatively near distances, with two energy minima; and 3) the net interaction is attraction (at moderate distances). However, if particles get very close (the distance of the inner net energy minimum) there is risk of coagulation, which results from interpenetration of the particles’ electrical environments.
Figure 6.12 illustrates two hydrophobic colloid particles at a stable distance from each other. (Note the stabilizing repulsive surface charges on surfaces of two particles; see Figures 6.9 and 6.12.) In this illustration the particles have positively charged surfaces, and thus conform to the requirement that they not attract one another. Closer inspection reveals a region that contains both positive and negative charges, immediately beyond the particles’ charged surfaces, that until now, has been referred to as a ‘diffuse area.’
The diffuse region of dispersed particles and the diffuse double layer model
What is this area of both negative and positive ions? First, nomenclature will be assigned. Ions that have the same charge sign as that of the particle surface (in this situation, positive) are called similions. Ions with the opposite charge sign as that of the particle surface (here, negative) are called counterions. The area just beyond the charged particle surface is called the diffuse region. The diffuse region then is composed of both similions and counterions.
Figure 6.12 Two charged colloidal particles at optimum separation distance
This figure illustrates various proximities of two charged particles in relation to net potential energy (PE). In (A), the particles are so close together they cannot practically be moved any closer. In order for the particles to get this close, a very large amount of energy would need to be applied in order to overcome the large repulsive force. In (B) interpenetration of the diffuse regions of the particles has occurred, meaning for a colloid, aggregation and coagulation. Though this is not desired, it can occur if the interparticulate distance is allowed to fall within the closer PE minimum. In (C), the particles are at an optimum distance for the dosage form, balancing attractive and repulsive PEs and residing in the outer PE minimum. In (D), the particles are too far apart to exert substantial interparticulate interactions.
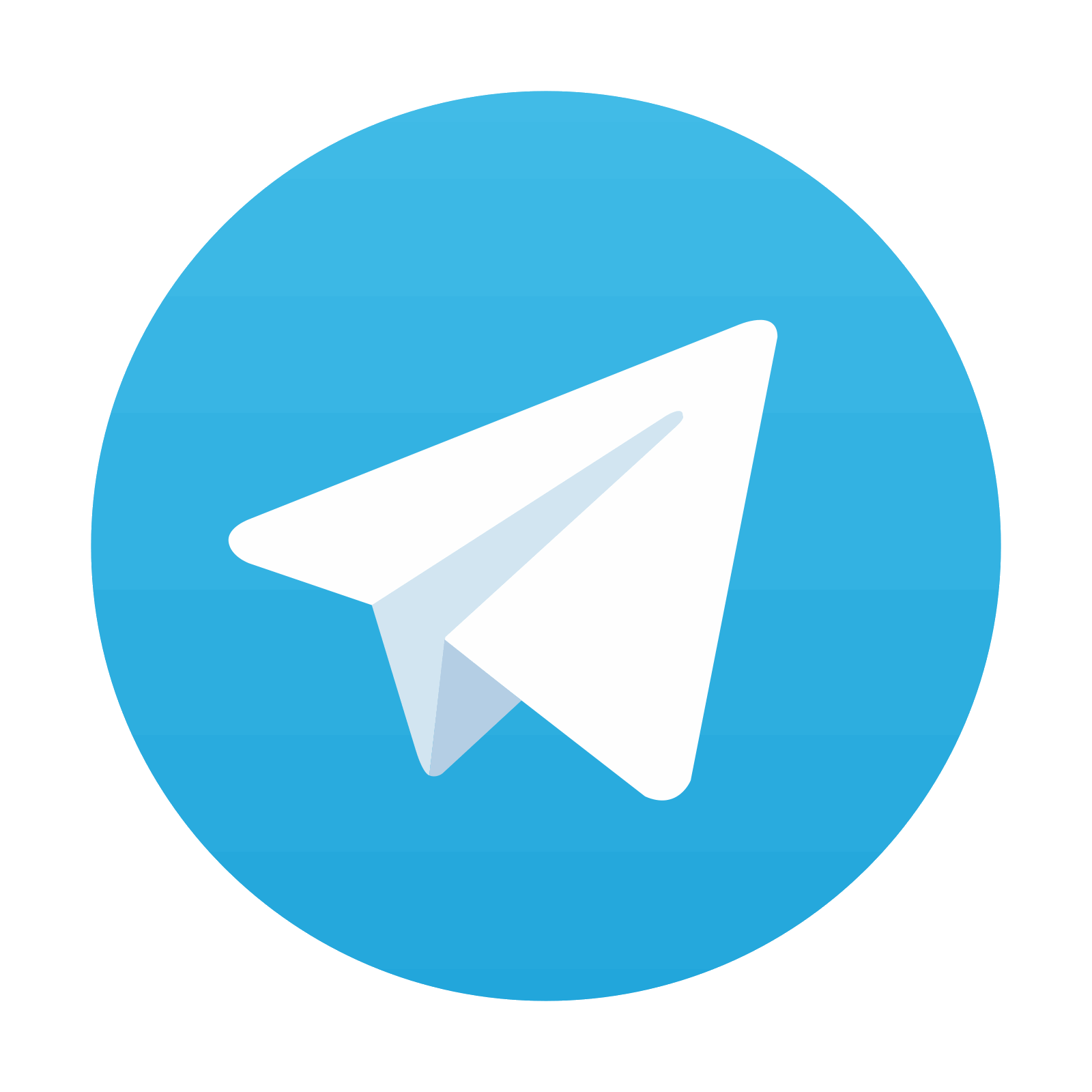
Stay updated, free articles. Join our Telegram channel
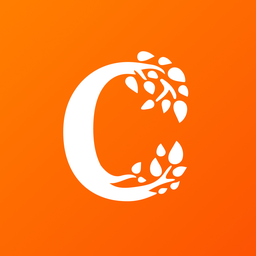
Full access? Get Clinical Tree
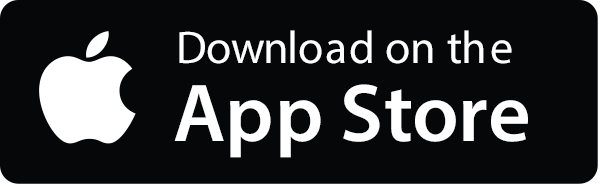
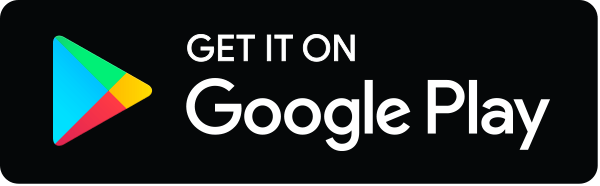