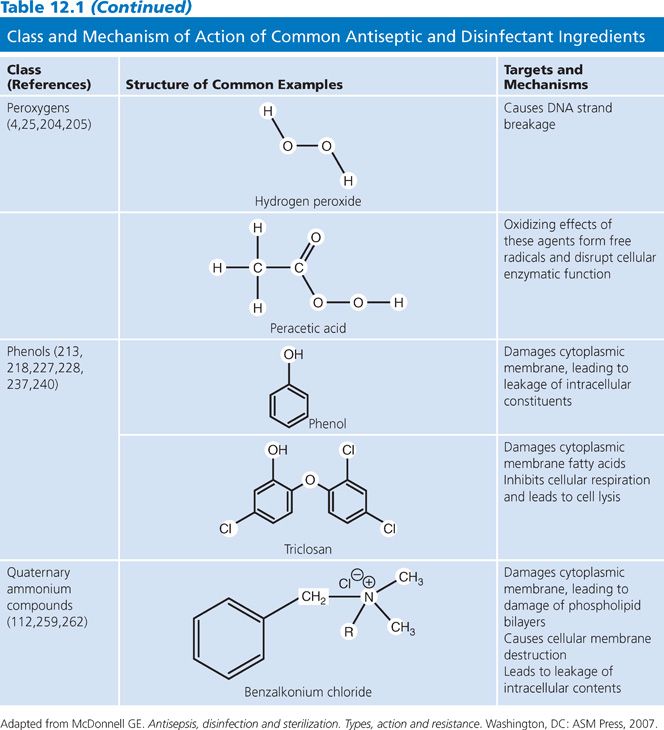
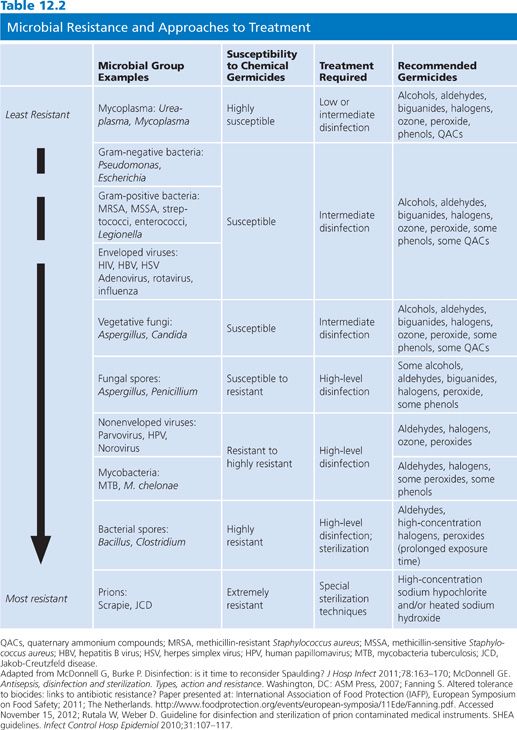
Sterilization processes must render surfaces and devices free of all living microorganisms, including spores. The sterility assurance level (SAL) defines the probability of survival of any viable organisms after sterilization treatment and is typically a −6 log10 or less than one in a million. Nonsterilization processes, although highly effective, produce instruments and surfaces that may yet contain some residual organisms and/or spores. It is important to appreciate that the use of chemical sterilants with shorter exposure periods will kill most microorganisms except large amounts of bacterial spores. Hence, sterilizing agents perform as high-level disinfectants in such situations. Unfortunately, some publications erroneously equate “disinfection” and “sterilization” or claim that items treated with short exposure times are “partially sterile.” The claim of chemical sterilization must be used to refer to absolute eradication of all types of microbes (4). Some agents that function as chemical sterilants include greater than or equal to 2.4% glutaraldehyde-based formulations, 0.95% glutaraldehyde with 1.64% phenol, 7.5% hydrogen peroxide, 7.35% hydrogen peroxide with 0.23% peracetic acid, 0.2% peracetic acid, and 0.08% peracetic acid with 1.0% hydrogen peroxide (see Table 12.2). These agents are most effective when cleaning precedes sterilization treatment and when standards for concentration, contact time, temperature, and pH are closely followed (4).
Antiseptics possess antimicrobial activity and may be used on living tissue to remove, inhibit the growth of, or inactivate microorganisms. The distinction between an antiseptic and a disinfectant is frequently ignored. However, the differences between a disinfectant and an antiseptic are important, and their applications are significantly different. A disinfectant is a chemical biocide used solely on inanimate objects or surfaces such as medical instruments or environmental surfaces; an antiseptic is to be used for living tissues. Some chemical agents, such as iodophors, can be active ingredients in either disinfectants or antiseptics. However, the precise formulations, patterns of use, and efficacy differ substantially. Consequently, products categorized as disinfectants should never be used as antiseptics and vice versa. Active ingredients used in antiseptics, including the iodine/iodophor group, biguanides (chlorhexidine), alcohols (usually ethanol or isopropanol), and phenolics (phenol and triclosan) have unique features, benefits, and drawbacks addressed in the remainder of this chapter.
SPAULDING CLASSIFICATION
The requirements for disinfection or sterilization of medical instruments, devices, or equipment can be more easily understood if these items are categorized based on the risk of infectivity involved with their use, as first suggested by Spaulding (5). The Spaulding classification system (Table 12.3) has been used since the 1950s by epidemiologists and microbiologists when discussing or planning strategies for disinfection and sterilization. The Centers for Disease Control and Prevention (CDC) has endorsed this system and has supported its use nationally and internationally (6).
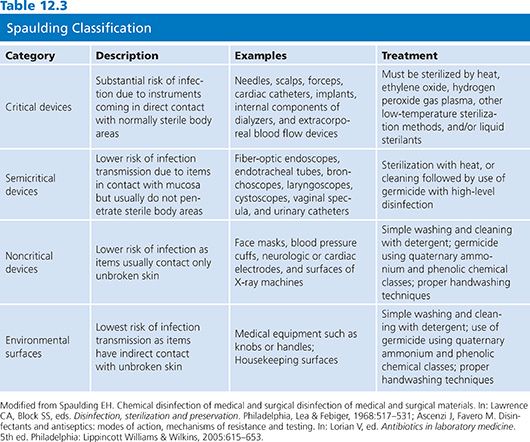
Spaulding (5) defined three surface categories for treatment: critical, semicritical, and noncritical. Critical instruments or devices present the greatest risk of acquiring infection if the item is contaminated with microorganisms at the time of use. These are instruments or objects that are introduced directly into normally sterile areas of the human body. Examples include needles, scalpels, forceps, cardiac catheters, implants, and also the inner surface components of extracorporeal blood flow devices such as of the heart–lung oxygenator and the blood-side of hemodialyzers. Critical instruments must be sterilized by heat (such as steam autoclave or dry heat), ethylene oxide, hydrogen peroxide gas plasma, other low-temperature sterilization methods, and/or liquid sterilants between patient procedures.
Instruments or devices classified as “semicritical” have a lower risk of infection. Examples include flexible fiber-optic endoscopes, endotracheal tubes, bronchoscopes, laryngoscopes, respiratory therapy equipment, cystoscopes, vaginal specula, and urinary catheters. This category of devices comes in contact with mucous membranes and do not ordinarily penetrate body surfaces. Sterilization of many of these items, although desirable and often cost-effective if steam autoclaves can be used, is not absolutely essential. Semicritical instruments or devices should, at a minimum, be subjected to high-level disinfection using a product, usually a liquid chemical germicide, available for use as a sterilant but applied for a shorter exposure time so as to function as a high-level disinfectant. This approach has broad-spectrum efficacy and can destroy some bacterial spores, most fungal spores, all ordinary vegetative bacteria, mycobacteria, small or nonlipid viruses, and medium-sized or lipid viruses. In practice, good cleaning followed by high-level disinfection provides the medical practitioner assurance that these semicritical items are relatively free of pathogenic microorganisms.
“Noncritical” instruments or devices come into direct contact with the patient but usually only with intact skin. This category includes face masks, blood pressure cuffs, most diagnostic electrodes, and certain surfaces of X-ray machines. Depending on the particular item and the nature and degree of contamination during prior use, simple washing or scrubbing with a detergent and warm water may be sufficient to safely allow reuse. Transmission of infectious agents to patients from environmental surfaces typically involves a vector, commonly hospital personnel (7–10). Studies have indicated that skin surfaces harbor organisms such as Clostridium difficile (11–14), methicillin-resistant Staphylococcus aureus (MRSA) (7–9), and antibiotic-resistant Enterococcus strains. Controlling transmission of these pathogens can be accomplished by disinfection of environmental surfaces and use of proper handwashing with products employing an effective active ingredient. Thorough handwashing, along with the use of effective antiseptics, is one of the most important facets of the current overall infection control strategy (15,16). Beginning with the work of Semmelweis in the 1840s and confirmed by the World Health Organization (17), effective handwashing alone reduces the transmission of infectious agents. When successful handwashing is achieved and paired with effective biocide(s), transmission of microbes can be further reduced (18).
Housekeeping surfaces have very low potential for cross-contamination between health care personnel, patients, and medical equipment and/or instruments. Safe levels of microbes can be achieved by keeping these surfaces clean by using water and detergent or a hospital-grade disinfectant. A detergent designed for general housekeeping purposes (as indicated on the product label) would be adequate as well. In instances of spilled blood or a potentially infectious body fluid or culture specimen, an intermediate-level chemical disinfectant should be considered to render the environmental surface “safe.”
Spaulding’s original classification may be modified to include additional environmental surfaces, which carry the least risk of disease transmission (19). These additional environmental surfaces may contribute to secondary cross-contamination by the hands of health care workers or by contact with medical instruments that will subsequently come into contact with patients. Surfaces in this category encompass only those that come into direct contact with intact patient skin. These environmental surfaces may be further divided into (a) medical equipment surfaces such as adjustment knobs or handles on hemodialysis equipment, X-ray machines, instrument carts, or dental units, and (b) housekeeping surfaces such as floors, walls, tabletops, curtains between hospital beds, window sills, and so forth. As with noncritical medical instruments, adequate levels of safety for environmental surfaces may be achieved by simple cleaning with a detergent and warm water, cleaning with soap and water, or application of an intermediate- to low-level chemical germicide. Controlling the level of contaminant on environmental surfaces is usually accomplished with agents belonging to the quaternary ammonium and phenolic chemical classes.
In current hospital disinfection practice, antimicrobial wipes are used both to clean and to disinfect environmental surfaces. Some antimicrobial wipes remove a large proportion of microorganisms from environmental surfaces. However, germicidal activity associated with wipes might be limited due to brief application times and repeated use on multiple surfaces (20). Inadvertently, wipes may transfer microbial contaminants to other surfaces after multiple wipings (20). Studies assessing the effectiveness of germicide-containing wipes have revealed variable benefit as biocides (21). For example, the crucial eradication of C. difficile spores was established using hypochlorite-soaked wipes; peroxide-, biguanide-, and ammonium chloride–soaked wipes were not effective (21).
Additional factors may also influence the efficacy of the disinfection and sterilization processes. These include prior cleaning of the item; amount of organic and inorganic material present on the item; type and amount of microorganisms present on the item; exposure time to the germicide and the concentration of the germicide used; the physical features of the item to be treated, that is, the presence of difficult-to-reach cracks and crevices that need to be reached during treatment; the existence of biofilms; and the importance of temperature and pH of the disinfection or sterilization product and the item(s) to be treated (4).
Recently, the Spaulding classification has been reaffirmed to be as useful today as when first developed in 1957. However, this schema may be an oversimplification as it does not consider problems noted earlier nor issues such as complicated medical equipment that may require unique considerations, such as heat sensitivity, and it does not address the concerns about killing certain difficult-to-treat infectious organisms (e.g., prions) (see Table 12.2). Therefore, in some situations, selecting the best method of disinfection is challenging, even after evaluating Spaulding’s criteria. The efficacy and testing for the required levels of disinfectants and sterilants needs to constantly be reassessed in current times to address newly identified and changing pathogens as well as newer environmental surfaces or items that are used in the health care setting (4,22).
REGULATION AND TESTING OF PRODUCT CLAIMS
In the United States, disinfectants are regulated by the Environmental Protection Agency (EPA) and the FDA under the Federal Insecticide, Fungicide, and Rodenticide Act (FIFRA) (4). Some states have additional regulations. Under FIFRA, any product must be registered following approval of its claim of safety and effectiveness. The EPA regulates low- and intermediate-level disinfectants, and the FDA regulates high-level disinfectants. This division of regulatory responsibility parallels the use of the products, that is, the EPA controls environmental surfaces and the FDA controls medical devices. Antiseptics are regulated solely by the FDA as drugs. In the European Union (EU), in 2013, an updated directive regulating biocides was implemented, establishing a two-step process of evaluation at the EU level and product authorization at the member state level (23).
Regulatory Agencies
Testing regimens used for product claims are defined by the EPA and FDA in the United States. In the EU, requirements are defined by directives for biocides, disinfectants, and antiseptics. The European Committee for Standardization Technical Committee (CEN/TC) sets the standards for products in the EU. In the United States, test procedures used for meeting data requirements for disinfectants generally are those formulated by the Association of Official Analytical Chemists (AOAC), the American Society for Testing and Materials (ASTM), and the International Organization for Standardization (ISO). Internationally, regulatory agencies in some countries (e.g., Canada, Australia, and Brazil) use these or similar test methods. In the United States, historically, hospital disinfectants, whether used for noncritical; semicritical; or critical devices, were regulated by the EPA under the authority of FIFRA (4). Additionally, some regulatory agencies have adapted different end points that one must meet to make a claim. For instance, in the United States, the quantitative tuberculocidal test requires a 6 log reduction to make a claim for tuberculocidal activity, whereas in the EU, using the same procedure, a 5 log reduction is required. Hence, the same product marketed in the United States and the EU may have differing requirements needed to claim activity.
Testing Regimens
Test procedures have been established for disinfectants and antiseptics under the auspices of AOAC. The test protocols are primarily for determining the effectiveness of these agents against bacterial spores, fungi, vegetative bacteria, and mycobacteria, and they serve to determine accurate product claims. In addition, potency of ingredients must be verified periodically due to potential impact of continued use as well as mixing with organic material, such as blood or soil, on product efficacy (24). Various approaches are available, ranging from simple laboratory evaluation to assessment under actual clinical conditions. These approaches allow not only for evaluation of biocide activity but also new product development, regulatory approval, labeling of products, and standardization. Efficacy claims as reported in the literature have validity under described test conditions. However, one must keep in mind the potential impact of environmental and institutional variability when generalizing these results to one’s local hospital practice.
The effectiveness of a disinfectant or antiseptic is assessed by testing in three stages. The first stage verifies whether a chemical has adequate antimicrobial activity; suspension tests are typically used (Table 12.4). The second stage is performed using methods that simulate real-life conditions. Ingredient activity with testing using standardized conditions, such as duration of contact time and temperature, are determined (e.g., surface testing). The third phase is “real life” or in-use tests. In-use tests also verify disinfectant effectiveness after employment for a typical period of time. These tests are discussed in detail in the following text.
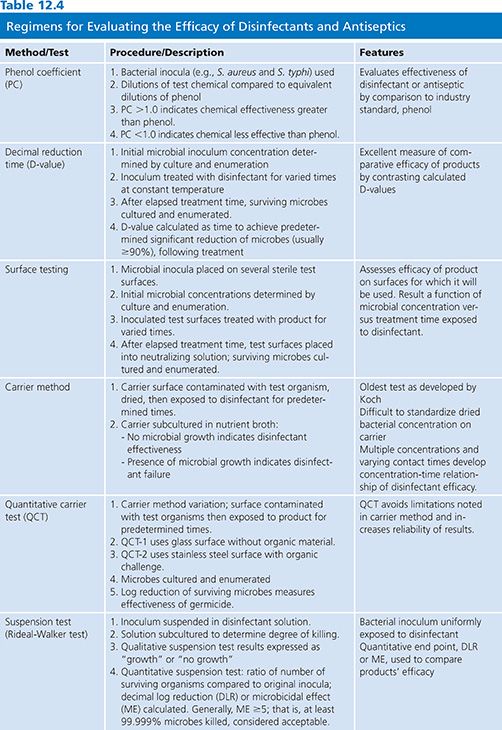
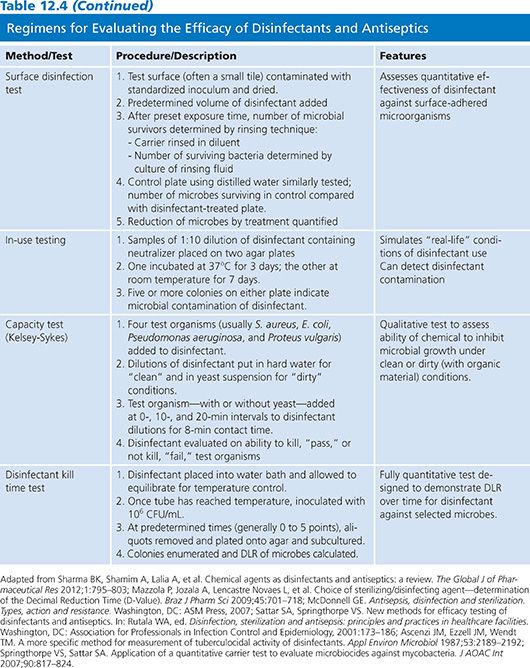
Laboratory test methods include determination of chemical uptake into cells, lysis of microorganisms and release of intracellular contents, alteration of cell wall permeability, alterations of cell membrane and metabolic function, enzymatic activity, disruption of biochemical pathways, and observation of microbial cell changes via microscopic visualization (25). Antimicrobial activity of the active ingredients is measured by determining the minimum inhibitory concentration (MIC), lowest concentration of the biocide that inhibits the growth of the microorganism, and minimal bactericidal concentration (MBC), the lowest concentration of the germicide that kills the organism. These test assays are modeled after those used for evaluating antimicrobial activity (see Chapter 3). Table 12.5 lists the MIC for several common microbes and germicide ingredients (26). Investigators often express the effectiveness of disinfectants in terms of decimal reduction time, or D-value, which is a commonly used measure of product efficacy. The D-value represents the time it takes, while at a constant temperature, to measure a predetermined significant reduction, usually 90% or greater, of the microbial load following treatment with a biocide (27). The microbicidal effect (ME) can also be determined for a product by subtracting the log number of microbes before and after treatment. The ME of 1 means there was 90% reduction of initial bacteria numbers, and ME of 2 equates to 99% killing. It is generally preferred that the ME is greater than or equal to 5, or that at least 99.999% microbes have been killed (24).
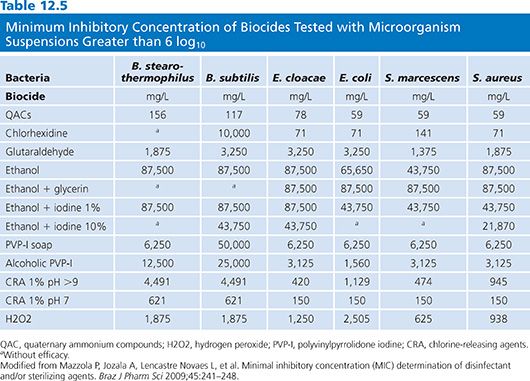
Ideally, a test protocol should simulate the conditions for which the product is intended. In vivo systems, involving human tissues or equivalent experimental models, and in situ systems, testing in real conditions, should be used when assessing antiseptics and disinfectants. For measuring in vivo or in situ activity, there are several protocols used to test formulations and preparations (see Table 12.4).
Suspension testing is a commonly used technique for assessment of efficacy of biocides (28). In this method, the test microbes are exposed to a series of chemical germicide dilutions and the MIC and/or MBC is determined. This method may be limited due to germicide interactions with organic or inorganic constituents in the growth media (e.g., halogens and aldehydes) or chemical agent interactions with the surface (e.g., chlorhexidine with certain fibers) (28). Analysis of the exposure time and the concentration of the biocide, along with other physical factors such as pH, temperature, and interfering substances (examples are organic materials such as serum or blood and inorganic materials such as hard water and soil), are crucial to determine the MBC and are practical considerations for use of the products in hospital settings.
Surface testing is necessary to assess the efficacy of the germicide on the surfaces for which they will be used. This is important for products to be used in hospital surfaces, including antiseptics, disinfectants, and sterilants. Typical methods used are carrier tests, simulated-use tests, and in-use testing. In the United States, stainless steel and porcelain surface items are used to perform carrier testing of products. The microbes are placed onto the item, with or without interfering substances such as soil, and then exposed to the germicide product. The test microbes are then assessed for survival via standard culture techniques. Simulated-use tests apply a sample inoculum of microorganisms to a surface to mimic actual use of the item and then wipe the surface with germicide. Recovery and culture then determines the survivability of the microbe. In-use testing is similar except that the assessment is performed in actual situations and conditions. This testing method may be recommended to evaluate the success of a product over time when used in hospital settings. The germicides must be assessed in-use with the most resistant organisms, such as spores, (“worst-case scenario”) to ensure actual sterilization is achieved. Detailed test methods, guidelines, and standards can be reviewed in McDonnell (28).
In-use testing for antiseptics requires in vivo assessment of products. Comparing in vivo studies is complicated because methods used vary greatly. For example, when studying different alcohol-based waterless antiseptic handrubs, methodologies vary as to (a) whether or not hands are purposely contaminated, (b) the volume of test substance used, (c) the time period the solution is in contact with the skin, (d) the methods used to recover organisms, and (e) the method of expressing efficacy. Several protocols have been used for determining the effectiveness of such antiseptics (29). Recent approaches have included a two-step process of in vitro testing followed by in vivo treatment evaluation (30). Alternatively, sequential in vivo application of two different antiseptics has been found to be highly effective (31). Product efficacy can be determined in vivo by using volunteers. In the Vienna test model, a variant of in-use testing, for example, a product is compared to standard disinfection assessed during parallel use with the same volunteers (32). This standardized approach allows for comparison of efficacy, by using experimental contamination of volunteers, and assessment of the survivability of the bacteria before and after disinfection. An exhaustive review of additional methodologic designs is provided by Hobson and Bolsen (33).
Numerous concerns about the traditional approaches to product testing have been posited (34–36). These concerns include lack of standardization of inocula, organisms’ resistance patterns, variations of the porcelain carriers used in the sporicidal tests, variations in the number of times the carriers are reused and processed, and uncertain relationship between the tested carrier surfaces and the surfaces to be disinfected in real-life situations. In response to these criticisms, alternative procedures have been proposed and adopted. The EPA adopted the use of a quantitative suspension test for measuring tuberculocidal activity of disinfectants (37) and modification of this methodology was adopted by ASTM (29). Further refinements have been developed to improve test reliability claims against mycobacteria (38). The updated quantitative carrier test (QCT) method has the following advantages: fully quantitative; minimizes the potential for false positives; measures kill under ideal and stringent conditions; increases the reliability of the results; can be applied to a wide variety of microorganisms, vegetative and spore forming; and eliminates the potential loss of organisms from wash-off during the exposure period. The QCT encompasses two separate parts, QCT-1 and QCT-2, which differ in the hard surface used (glass vs. stainless steel) and the type of organic soil challenge incorporated into the test. QCT-1 tests the disinfectant under more ideal conditions, using a glass surface, and without including organic soil, while QCT-2 tests the disinfectant under less ideal conditions. The latter is achieved by use of stainless steel (a surface that can have more variability), less disinfectant, and an organic challenge added to the suspension. QCT-1 has been validated for use with vegetative bacteria, including mycobacteria, bacterial spores, and fungi, whereas QCT-2 has been validated for use with viruses and protozoans in addition to vegetative bacteria, mycobacteria, bacterial spores, and fungi. The data obtained from these procedures allows one to calculate a log reduction value for the potency of the germicide. Each regulatory agency may choose to set their end point, the SAL, for determining product efficacy.
EPA developed a method for evaluating chemical germicides for virucidal activity, a method also accepted by the FDA for those products under its authority. Claims made based on testing against specific viruses, such as norovirus and hepatitis B and hepatitis C viruses, have been allowed based on testing using the surrogate viruses feline calicivirus, duck hepatitis virus, and bovine diarrhea virus, respectively (39).
Although some chemical germicides maybe labeled as sterilants, guidelines from the CDC, FDA, Association for Professionals in Infection Control and Epidemiology (APIC), and other professional organizations do not recommend the use of these germicides for sterilization unless there are no other available products. Unlike terminal sterilization processes (such as steam, ethylene oxide, and peroxide gas plasma), where the items can be maintained in a microorganism-free state by packaging after sterilization, items so treated using liquid processes cannot be maintained in a sterile state when they are removed from the solution. Sterilization claims can be obtained for the liquid processed products using the AOAC sporicidal test (40). Humphreys’ (41) report identifies the currently available methodologies and standards for sporicidal products.
ACTIVE INGREDIENTS
The active ingredients in antiseptics and disinfectants achieve their effects through interactions with the microorganism cell surface followed by penetration into the cytoplasm and action on cellular targets. The variable response to these products may be due to composition of the cell surface, change in the environment, and increasingly identified resistance to germicides. The following sections describe mechanisms of action and indications for commonly used products (summarized in Table 12.1; Fig. 12.1) (25,42).
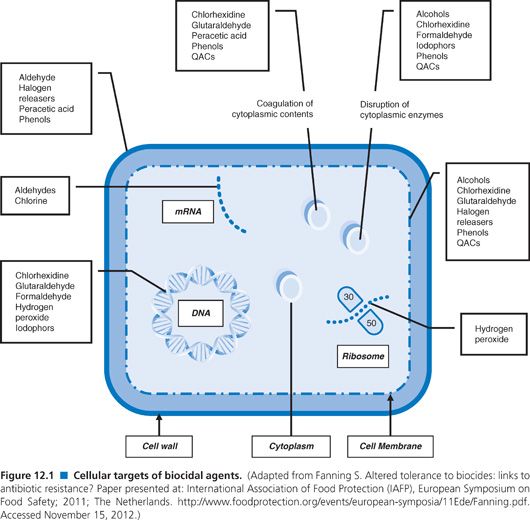
BIGUANIDES
Chlorhexidine
Biguanides are compounds that contain the C2H5N7 component. Chlorhexidine, a substituted biguanide, has a high degree of antimicrobial activity, low mammalian toxicity, and the ability to bind to the stratum corneum layer of the skin and to mucous membranes (43). The bactericidal activity of chlorhexidine is more potent than that of monomeric biguanides, hence is the only biguanide discussed in detail in the following text (44). These unique characteristics make it particularly attractive as an active ingredient in antimicrobial skin preparations. Chlorhexidine’s general chemical structure is shown in Table 12.1.
Practically insoluble in water, chlorhexidine reacts with acids to form salts with varying degrees of solubility. Recognized as an effective antimicrobial, chlorhexidine is the active ingredient in a number of products: preoperative skin preparations, surgical hand scrubs, health care personnel handwash products, skin cleansers, acne creams, oral products (such as mouthwashes), burn ointments, and wound protectants; it has also been incorporated into products as a preservative.
Mechanism of Action
The activity of chlorhexidine relates to the interaction of the germicide with the cell surface (see Table 12.1 and Fig. 12.1), causing changes in the lipids in the cell membrane (45–47). Changes in the integrity of the membrane result in loss of membrane function. Experiments using 14C-chlorhexidine indicate that chlorhexidine rapidly enters the cells of both bacteria and yeasts (48,49). The bacterial cell wall is generally negatively charged and is rapidly neutralized in the presence of chlorhexidine (43), indicating the reaction of chlorhexidine with the cell membrane. The outer membrane structure in gram-negative bacteria is stabilized by the interaction of divalent cations and the negative charges associated with lipid A of the lipopolysaccharide (LPS) in the cell membrane. It is hypothesized that polycationic molecules, such as chlorhexidine, can promote their own uptake by displacement of divalent cations associated with the LPS. Alterations in the LPS can lead to changes in susceptibility to antimicrobials (50), whereas disruption of the cell membrane results in leakage of cellular material from the cell. The leakage in Escherichia coli and S. aureus is biphasic, depending on chlorhexidine concentration (47).
At low concentrations (up to 200 μg/mL), chlorhexidine inhibits membrane enzymes and promotes leakage of cellular constituents. Cells treated with bacteriostatic concentrations lost up to 50% of their K+ but can recover upon neutralization of excess chlorhexidine (43,51). At higher concentrations, cytoplasmic contents are coagulated (52) and cells begin to leak higher molecular weight molecules such as nucleotides (43). Uptake of bis-(1,3-dibutylbarturic acid) trimethane oxonal and propidium iodide, which reflect membrane potential and membrane integrity, respectively, was directly related to chlorhexidine concentrations (0.003 to 0.3 mmol−1), indicating changes in membrane structure with increasing concentrations of chlorhexidine (53).
The effect of chlorhexidine on mycobacterial cells is bacteriostatic, not bactericidal. The treatment of mycobacterial cells with ethambutol (an antituberculosis drug known to inhibit the synthesis of specific components of the mycobacterial cell wall) potentiates the activity of chlorhexidine against Mycobacterium spp, indicating that chlorhexidine needs access through the cell membrane to exert its activity (54).
For yeast, chlorhexidine can similarly damage the cytoplasmic membrane. Treatment of Saccharomyces cerevisiae cells with chlorhexidine resulted in the release of pentose indicating increased oxidative stress and cellular leakage from these organisms (55). In Candida albicans, sublethal concentrations of chlorhexidine resulted in loss of cytoplasmic components and coagulation of nucleoproteins (56) and prevented the outgrowth but not the germination of spores (57).
The general mechanisms of chlorhexidine can be summarized as follows:
■ Damage to cell membrane by interaction with lipid layer
■ Leakage of cellular constituents at low-level exposure
■ Precipitation of nucleic acids and proteins at high-level exposure
■ Coagulation of intracellular and disruption of enzymatic pathways at high-level exposure
Spectrum of Activity
A comprehensive review of in vivo and in vitro effectiveness of chlorhexidine activity has been reported (43). MICs for bacteria and fungi are presented in Tables 12.6 and 12.7, respectively. These data indicate the broad spectrum of activity of this ingredient, which is effective against gram-positive and gram-negative bacteria, yeasts, and molds.
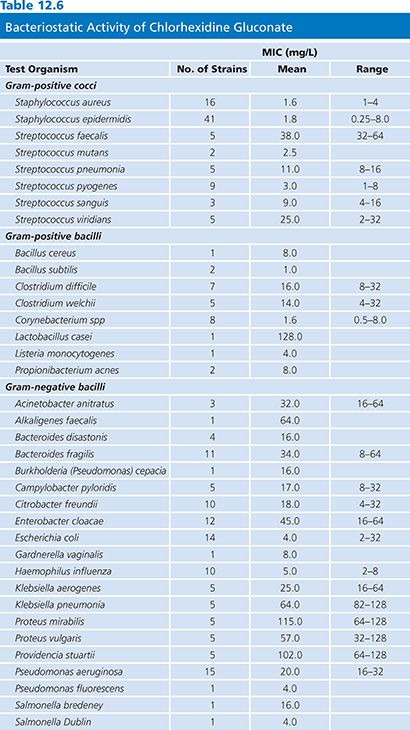
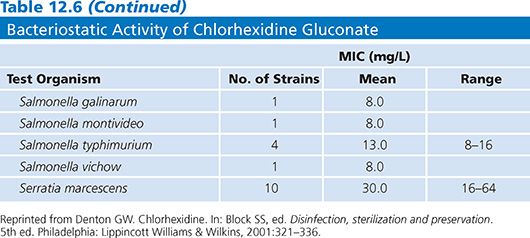
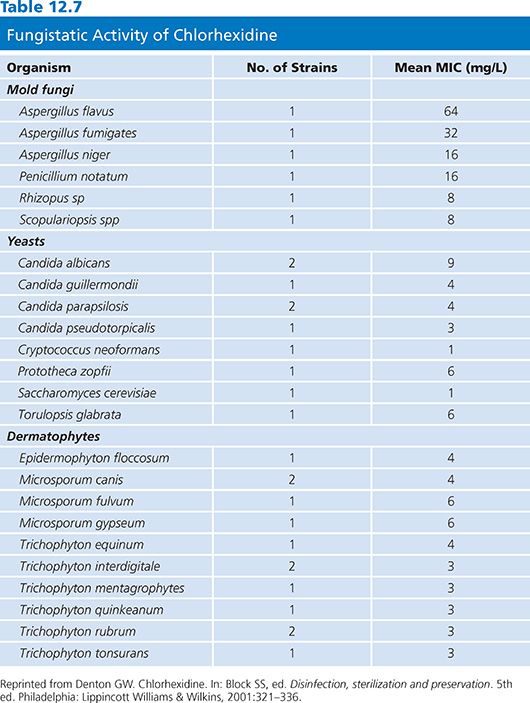
Studies of a 0.05% chlorhexidine solution confirm the rapid inactivation of gram-positive and gram-negative bacteria, yeasts, and molds (43). Following a 10-minute exposure to this solution at 18°C to 21°C, only resistant microorganisms, such as Pseudomonas stuartii, Streptococcus faecalis, Bacillus subtilis, and C. difficile, did not show substantial reduction. The MIC values for B. subtilis and Clostridium spp (see Table 12.6) are relatively low, indicating the bacteriostatic effect on these organisms. All of the other bacterial species tested showed a greater than 2 log10 reduction in 10 minutes. Gram-negative bacteria were generally more susceptible (4.0 to 6.7 log10 reduction) than gram-positive bacteria (2.1 to 5.8 log10 reduction) under the conditions tested (58). Treatment of E. coli and Klebsiella pneumoniae with sublethal concentrations of chlorhexidine resulted in lower pathogenicity of these organisms when injected into mice (58). With the exception of the more resistant organisms listed earlier, among the gram-positive bacteria, the cocci (2.1 to 5.8 log10 reduction) were generally more resistant than the gram-negative bacilli (3.6 to 4.8 log10 reduction).
Data indicate that chlorhexidine may be capable of inhibiting spore growth and germination but that it does not kill the spores. As shown in Table 12.6, the MIC for spores is quite low. Data indicate that both B. subtilis and C. difficile spores show less than 1 log10 reduction when exposed to a 0.05% solution of chlorhexidine. Sporicidal activity can be achieved with chlorhexidine at higher temperatures (57).
Chlorhexidine has antiviral effects due to its interaction with viral membrane lipids. Viruses can be grouped into those with an outer lipid membrane composed of lipoproteins and glycoproteins around the protein coat (enveloped viruses) and those that are nonenveloped (i.e., lack a lipid membrane). Due to its solubility characteristics, chlorhexidine is more active against enveloped viruses with a lipid membrane (Table 12.8), such as HIV. Studies confirm the effectiveness of chlorhexidine against HIV. Preparations containing chlorhexidine (a 4% and a 0.5% solution in 70% alcohol) were completely effective against HIV-1 after 15 seconds of contact. An aqueous solution of 0.05% was equally effective in 1 minute (59). Aqueous chlorhexidine gluconate at 0.2% was effective but at 0.02% was not effective (60).
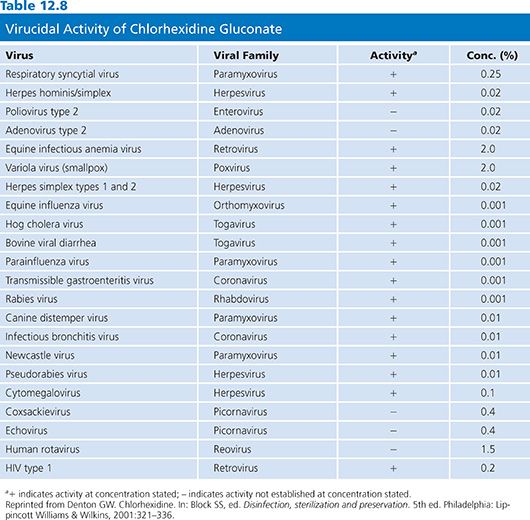
In vivo studies using chlorhexidine formulations provide further evidence of the activity of this germicide. The chemical components and the solubility of various chlorhexidine salts can make a difference in the activity of the formulation. When used in the presence of organic ions such as soaps, sodium lauryl sulfate, sodium carboxymethyl cellulose, alginates, and some dyes, chlorhexidine may be less effective. Many of these compounds are commonly used in formulations for antiseptic preparations. Although there may be no visual evidence of incompatibility, the chlorhexidine can be incorporated into micelles and not be readily available to interact with microorganisms (43). The addition of anionic thickeners or emulsifiers also serves to inactivate the long-lasting antibacterial activity (persistence) of chlorhexidine (61).
Chlorhexidine has been incorporated into detergent and alcohol preparations, powders, mouthwashes, and polymeric preparations for a variety of uses. Given its ability to bind to the stratum corneum layer of the epidermis, chlorhexidine exhibits persistence and substantivity; that is, it exerts its effect long after it has been used, and the more it is used, the greater the reduction in the resident flora of the skin. This activity is especially relevant for its use as a surgical scrub and in surgical site preparation.
As a surgical scrub, chlorhexidine has been prepared as 4% and 2% aqueous solutions as well as alcohol-based formulations of different concentrations. Chlorhexidine is effective in removing transient microflora from artificially contaminated hands (62). The effects are immediate and long-term (persistent or residual) on resident microflora (63). A study examining the difference between aqueous and alcohol preparations found that a formulation containing 1% chlorhexidine in 61% ethanol resulted in significantly greater microbial reduction than a 4% aqueous solution or the 61% ethanol vehicle alone (64). When used as a health care personnel handwash, chlorhexidine has typically been employed as the active ingredient in aqueous or alcohol-based solutions. Comparison of four chlorhexidine formulations (two 4% aqueous formulations, a 2% aqueous chlorhexidine formulation, and an alcoholic 0.5% chlorhexidine formulation) versus a nonantiseptic soap revealed that after the initial wash with each, there was no difference in microbial reductions. However, as more washes were performed, there was a significant difference between the four chlorhexidine-containing formulations as compared to the soap, although there was no difference between the chlorhexidine formulations (65).
Incorporation of chlorhexidine into surgical skin prep (2% chlorhexidine in 70% isopropyl alcohol) has proven to be effective. The chlorhexidine solution significantly reduced abdominal and inguinal microbial counts from baseline for up to 24 hours. It was more persistent in the abdominal region than were two other preparations containing isopropyl alcohol or chlorhexidine alone (66).
Chlorhexidine-containing powders used in routine umbilical decontamination in a neonatal unit controlled MRSA (67). Several studies have been done comparing infection rates when chlorhexidine gluconate and povidone-iodine were used as skin preparations in patients with central venous catheters. Comparison of catheter-related bloodstream infections indicates that chlorhexidine gluconate was superior; however, all the studies were limited by the small numbers of subjects (68–70). A meta-analysis indicates that chlorhexidine reduced the risk of catheter-related bloodstream infections by 50% (71). Several studies have assessed whether catheters impregnated with chlorhexidine and silver sulfadiazine (CHX-SS) reduced catheter-related bloodstream infections. When comparing patients with short-term indwelling catheters (median duration of 6 days) and those with long-term indwelling catheters (median duration of 12 days), a meta-analysis indicated that those in the short-duration group using CHX-SS catheters had a significant reduction in the incidence of catheter-related bloodstream infections, whereas the longer duration use group showed no difference between the CHX-SS catheters and standard catheters (72). In vitro studies with CHX-SS supported the data from the in vivo studies (73).
The incorporation of chlorhexidine into oral solutions goes back over 30 years (74), including mouthwash, varnish, and slow-release dental treatments. Chlorhexidine in varnish used on orthodontic patients helps prevent caries. Newer techniques such as epifluorescence microscopy can verify the dental effectiveness of chlorhexidine and other antimicrobial ingredients (75). Data indicate that there is a significant reduction in the level of Streptococcus mutans 1 week after the application of a sustained-release varnish. The decrease in S. mutans was measured for up to 3 weeks after the application. However, chlorhexidine-coated toothbrushes used for 30 days did not exhibit less bacterial contamination than untreated toothbrushes (76). A recent study compared the efficacy of chlorhexidine and a garlic extract in reducing colony-forming units of S. mutans. Although the chlorhexidine was beneficial, the garlic extract was more effective. It seems unlikely that the general public would be willing to perform regular garlic extract rinses, however (77). Studies have also shown high activity against typical oral flora when exposed for 1 minute to chlorhexidine digluconate 0.01% to 0.5% and no evidence of significant antimicrobial resistance was detected (78). Chlorhexidine may reduce ventilator-associated pneumonia (79) and possibly postdental implant bacteremia (80). Even with this known effect, current literature does not support a role for chlorhexidine rinses to prevent endocarditis in high-risk patients but instead recommend consistent comprehensive oral hygiene (81).
HALOGEN RELEASERS
Halogen releasers function as oxidizing agents, with bromine, chlorine, and iodine commonly used as disinfectants and antiseptics. Only certain halogen-containing species with iodine and chlorine are active antimicrobials due to their solvency and chemistry. Only these halogen releasers will be reviewed in this section.
Iodine and Iodophors
Iodine in the form of a tincture (alcohol extract of a low volatility substance) has been used since the early 1900s as a preoperative skin preparation. Cutaneous toxicity limited the use of iodine, whether in an aqueous or tincture preparation. The use of iodophors, which are complexes of iodine with a carrier such as polyvinylpyrrolidone (PVP) (see Table 12.1), allows for the slow release of free iodine, the active species, thereby reducing the preparation’s toxicity and staining without limiting the antimicrobial activity. Iodophors have been used as both antiseptics and disinfectants in the form of solutions, ointments, and aerosols. Iodophors are classified as low- or intermediate-level disinfectants (82) because they are not sufficiently sporicidal in an appropriate short application time. The amount of free iodine present in iodophors is difficult to determine. Concentrated solutions contain less free iodine than those that are diluted (83), and free iodine is the active species, whereas the PVP or other molecules with which the iodine is complexed act as carriers. A comparison of several commercial formulations of 10% polyvinylpyrrolidone iodine (PVP-I) had two orders of magnitude range of free iodine, hence a wide range of antimicrobial activity (84). Improperly diluted ionophore formulations allow for less free iodine and lower antimicrobial effects (85). This was the presumed cause of the outbreak of bacteremia associated with Pseudomonas aeruginosa when using a contaminated 10% PVP-I solution (86). Today, the primary use of iodophors is as an antiseptic for surgical scrubs and skin preparations.
Mechanism of Action
The killing effect of iodine and iodophors is related to the concentration of the free molecular iodine, whose concentration correlates with antimicrobial activity (83,87). The exact mechanism of action of iodine is not known. Its ability to penetrate the cell wall rapidly may be the primary mode by which it exerts its antimicrobial activity (see Table 12.1 and Fig. 12.1). Iodine can cause disruption of cells or cellular components as follows:
■ Reactions with N–H groups of amino acids during which N-iodo compounds are formed, resulting in destruction of proteins
■ Irreversible oxidation of -SH groups of cysteine, leading to loss of protein disulfide bonds (84,88)
■ Iodination of phenolic and imidazolic groups of the amino acids tyrosine and histidine and iodination of the pyrimidine derivatives of cytosine and uracil, leading to steric hindrances in hydrogen bonds and denaturation of DNA
■ Iodine binding to unsaturated fatty acids, altering the physical properties of lipids and hence lipid-containing membranes
For a more detailed description of the biochemical properties of these agents, the reader is referred to Gottardi (84).
Chlorine
The antiseptic- and disinfectant-oxidized chlorine compounds are formed in water. These include elemental chlorine, hypochlorous acid, and hypochlorite ion; the latter being the most widely used form. The antimicrobial action of available chlorine depends on the concentration, temperature, and pH of the solution; higher pH solutions have diminished antimicrobial activity. Many chlorine products have an irritating and corrosive nature, limiting their clinical use.
Disinfection of water is a common use of chlorine products and historically has controlled widespread waterborne diseases such as cholera and typhoid. Hypochlorites are used as environmental surface disinfectants but residual chlorine may need to be removed to prevent corrosion (28).
Mechanism of Action
Chlorine causes oxidation of proteins and lipids, thereby damaging the cell wall and membrane. Hypochlorous acid is the most active product and even at a neutral pH penetrates the outer cell layers. The moiety disrupts oxidative phosphorylation and other enzymatic activities of the cell. This germicide affects membrane-bound as well as cellular functional proteins, particularly those with sulfhydryl bonds (28), similar to the iodophors. Hypochlorous acid suppressed E. coli growth via complete inhibition of DNA synthesis and viral RNA is degraded into fragments (89). Increased bacterial spore and fungal cell wall permeability has been observed, which causes better biocide penetration and microbe killing (89).
The general mechanisms of chlorine can be summarized as follows:
■ Damage to cell membrane by oxidation of proteins and lipids
■ Inhibition and degradation of DNA and RNA
■ Increased spore and fungal cell wall permeability and subsequent microbe death
Spectrum of Activity
The antimicrobial activity of the halogen releasers and iodine and iodine-containing formulations is similar to chlorine-releasing compounds; these formulations have broad-spectrum activity against gram-positive and gram-negative bacteria, mycobacteria, fungi, and viruses (88). As with most chemical germicides, the activity of iodine and chlorine are dependent on their concentration, the formulation, the temperature, the growth conditions of the microorganism, and the presence of organic and inorganic material.
The sporicidal activity of iodine compounds is significantly less than chlorine-releasing compounds. A concentration of 2,000 to 5,000 ppm potassium iodine is necessary to produce a 5 log10 reduction in B. subtilis spores within 5 minutes. Iodine solutions are somewhat effective in destroying spores of Bacillus anthracis, B. subtilis, Bacillus megaterium, Bacillus mesentericus, Clostridium tetani, and Clostridium welchii (90–92) with prolonged application. Generally, disinfectant formulations of iodophors are weakly sporicidal with prolonged application, whereas antiseptic-containing iodophors are not sporicidal (93).
The activity of iodine against Pseudomonas spp and Legionella pneumophila isolates from a water system was dramatically different if the organisms were grown in water versus rich medium. Water-grown organisms proved more resistant to the iodine (94,95). Studies have also shown that iodine-containing formulations are more effective against vegetative organisms than are chlorine-based formulations (88).
Antimycobactericidal activity of iodine and iodophors are inconsistent (96–98). The following data exemplify the lack of consistency regarding the tuberculocidal activity of iodine and iodophors; hence, these compounds are not considered high-level disinfectants. Some tests showed that very low concentration of free iodine (<0.1%) killed M. tuberculosis with a 5-minute exposure at room temperature (96). In other tests, however, several commercially available iodophor formulations were not effective in killing two strains of M. tuberculosis after a 30-minute exposure (97). A comparison of two different iodine formulations, povidone-iodine (1% titratable iodine) and an iodophor (0.008% titratable iodine), demonstrated that the iodophor was ineffective in killing greater than a 3 log10 CFU M. tuberculosis after 1-minute contact in suspension or dried on a carrier. The povidine-iodine was effective in producing more than a 3 log10 reduction in a suspension test but not when the organism was dried on carriers in the presence of sputum (98). The iodophor product was also ineffective against Mycobacterium smegmatis (99). As a result of these inconsistent tuberculocidal effects, these compounds are not classified as high-level disinfectants.
Chlorine, iodine, and iodine-containing products have good fungicidal and fungistatic properties. They are effective against species of Trichophyton, Monilia, Epidermophyton, Torula, and other genera (88,100).
Iodine and iodophors are effective against a wide range of viruses, including enteroviruses and polio, herpes, vaccinia, rabies, and tobacco mosaic viruses (101–103), with inactivation concentrations of 75 to 150 ppm for polio type 1, coxsackie B1, adenovirus 2, vaccinia, herpes, influenza A, and HIV-1 viruses and 5,000 ppm for feline parvovirus. Complete inactivation of cell-free HIV was accomplished using 0.5% PVP-I with a 30- to 60-second exposure. Quantitative suspension testing has shown virucidal efficacy of povidone-iodine disinfectants against vaccinia, polyomavirus SV40, adenovirus and poliovirus, although more than 60 minutes of exposure was required for the most resistant poliovirus species (104). Some preparations of PVP-I have proven to be active against hepatitis B virus and enteroviruses as measured by the morphologic alteration test, while other preparations had no activity at all (105). In accord with the observation for Pseudomonas spp, this variability may be attributable to the amount of free iodine in the preparation.
Iodine-containing solutions have been primarily used as skin preparation/degerming solutions and surgical hand scrubs. Chlorine antiseptics are not used commonly due to skin irritation. In general, most iodine-containing skin prep and surgical scrubs show rapid reduction in the resident flora of the skin; however, they do not show residual activity. Studies have shown them to be inferior to chlorhexidine gluconate (106). Utilizing the Vienna test method, a 3 log10 reduction was achieved after a 2-minute wash with PVP-I; the same effect was achieved with chlorhexidine gluconate after a 1-minute wash (32). These results were confirmed comparing 14 different iodine preparations (107). In general, PVP-I solutions are more negatively affected by the presence of organic material than are chlorhexidine formulations (108,109).
ALCOHOLS
Alcohols have been used for disinfection of both skin and surface since antiquity (110). In Europe, the use of ethanol as a skin antiseptic has been the standard (110), while in the United States, ethanol is often replaced by antiseptic chemicals such as chlorhexidine, povidone-iodine, and triclosan. Alcohol preparations have been used as hygienic hand disinfectants, surgical hand disinfectants, and skin disinfectants, each having its own set of requirements. In addition, alcohol is often a constituent of combination skin disinfectants. Health care workers should be aware that anionic additives in hand disinfectants containing alcohol may inactivate antibacterial activity and persistence of chlorhexidine (61).
Alcohols have the general chemical formula R–OH, where R can be any organic structure such as an alkyl or benzyl molecule (see Table 12.1). The –OH group is the reactive species and its reactivity is influenced by the attached R group.
Alcohols have been commonly used as hard-surface disinfectants because of their general antimicrobial properties. However, volatility and flammability can be an issue when using alcohols in this manner. The flash points of ethanol and propanols are below 15°C, requiring caution in their use. On the other hand, short-chain alcohols can be suitable as disinfectants because they have unlimited solubility in water, have low toxicity, are fast acting, and are microbicidal. Low-weight alcohols possess less surface tension than water and thus have better wetting characteristics. This is important for antiseptics used on skin or for surface disinfectants used on nonuniform surfaces (110).
Regardless of the application, the order of antibacterial effect of alcohol isomers is n-primary > iso-primary > secondary > tertiary. This review is confined to the use of alcohols as antiseptics (i.e., their use on skin), as that is the primary use in health care today.
Mechanism of Action
It is generally believed that alcohols, like most chemical germicides, act at multiple sites of the cell. The primary mode of action is related to coagulation/denaturation of proteins and solubility of the alcohols in lipids (111,112) (see Table 12.1, Fig. 12.1). In the absence of water, coagulation will not occur. For example, 70% ethanol is more effective than 95% ethanol as a germicide because higher concentrations of alcohol may deprive the bacterial cell of water, thereby inducing an impermeable cell membrane. This activity prevents the penetration of alcohol into the cell (110). Hence, with dry cells, water is needed for antimicrobial activity, though this is not true for moist cells.
Coagulation of cell wall and cytoplasmic proteins occurs after exposure to alcohol disinfectants but does not appear to affect nucleoproteins (113). Coagulation of enzymatic proteins leads to loss of cellular function but there is no specificity with regard to which enzymes are affected. In addition, as the chain length of the alcohol increases, there is further impact on diminishing enzyme function (114). Bacterial enzymes in the cell wall are more easily inactivated than intracellular enzymes (115). Microbe cell lysis following alcohol exposure is likely due to disruption of the cell membrane (110,116,117).
The general mechanisms of alcohols can be summarized as follows:
■ Coagulation/denaturation of proteins and lipids
■ Water-dependent activity due to water requirement to achieve cell membrane permeability
■ Cytoplasmic components coagulate after intracellular concentration
■ Disruption of cell membrane followed by cell lysis
Spectrum of Activity
A review of the in vitro activity against bacterial spores indicates that alcohols are considered to have little killing effect. As a matter of routine procedure, spore preparations are often kept in 95% ethanol to prevent them from contamination by other vegetative organisms. However, other data indicate that some sporicidal activity may be found and is related to the alcohol itself, its concentration, and the species of microorganism (110).
A number of studies document the activity of alcohols against gram-positive and gram-negative organisms (118–120) and against multidrug-resistant pathogens (121–123). Tables 12.9 and 12.10 summarize the antimicrobial activity of a variety of alcohols against common microorganisms using carrier and suspension testing methods.
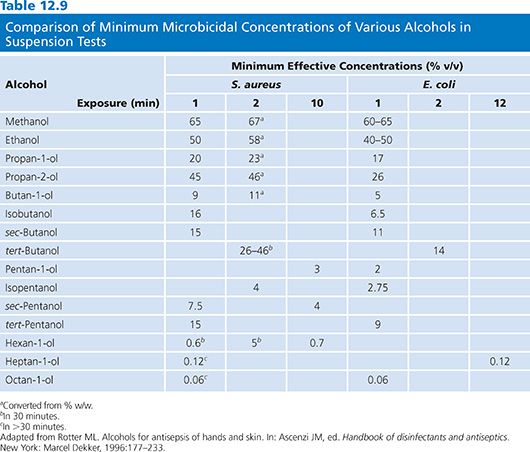
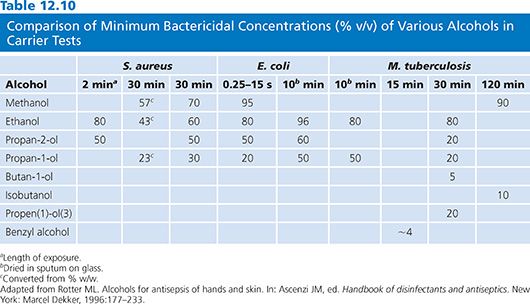
Ethanol is bacteriostatic and inhibits spore growth at concentrations around 10%, is bactericidal at 30% or more, but loses its activity at 90% or more, indicating the need for water for biocidal activity to kill S. aureus as well as other bacterial species (118,124).
Alcohol has in vitro activity against M. tuberculosis and a variety of fungi (110,119,125). Clinical isolates of Mycobacterium have been shown to be resistant to 75% alcohol (the alcohol type was not identified). Mycobacterium chelonae and Mycobacterium nonchromogenicum showed prolonged survival in 75% alcohol (126). Water content is necessary for the effectiveness of this antiseptic (Table 12.11).
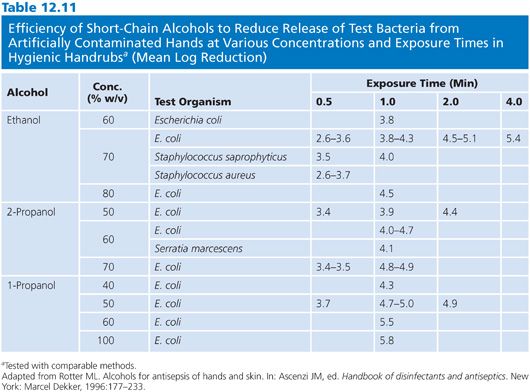
The fungicidal activity of short-chain alcohols, mainly ethanol, is well established (110). Most are killed within short exposure periods (5 to 60 minutes) at concentrations ranging from 35% to 96%. Candida spp and Aspergillus niger appear to be very susceptible, with a minimum effective concentration of 35% at an exposure time of 1 minute.
As with other chemical agents, the virucidal activity of alcohols is generally related to the presence or absence of a lipophilic envelope. Among the enveloped viruses, methanol, at concentrations of 20% to 80%, inactivates viruses in 15 minutes to 24 hours (127,128). Ethanol at concentrations ranging from 50% to 60% inactivates the same viruses in 1 hour (110). The propanols seem more active, since they require lower concentrations to have the same effect with similar exposure times (110,129,130).
The nonenveloped viruses such as picornaviruses, however, are more difficult to kill with longer chain alcohols than with ethanol. Seventy percent ethanol was effective in 2 minutes against poliovirus type 1 (131), though at 25% concentration, a 240-minute exposure was necessary for killing. Ninety-five percent 2-propanol was not effective after exposure for 10 minutes against this virus. Coxsackievirus type B was not killed by 95% 2-propanol after 10 minutes of exposure (130). Echovirus type 6 was inactivated with 50% ethanol in 10 minutes, whereas 90% 2-propanol was not effective. Seventy percent ethanol and 45% 2-propanol were not effective against hepatitis A after 1 minute of exposure (132) but hepatitis B, which is normally considered resistant to many chemical germicides, was relatively sensitive to both alcohols (133,134).
The microflora of the skin can be divided into two groups: the resident flora (more difficult to remove) and the transient flora (easily removed). The resident microflora also replicate on the skin. Health care personnel handwash preparations are designed primarily to remove the transient organisms, whereas surgical scrubs and skin prepping formulations are directed more at reducing the resident flora. It has been demonstrated that although bacteria on a patient’s skin are the main cause of surgical site infections, it is important for health care personnel to further reduce the risk of infection by careful hand hygiene (110). Studies demonstrate that alcohol rubbed on the skin reduce the microbial flora as effectively as a 6-minute scrub with water. Unlike other antiseptics (e.g., chlorhexidine and triclosan), alcohols do not have any residual or substantive properties. Mixtures of alcohols and agents such as chlorhexidine combine the rapid effect of the alcohol with an ingredient with substantive activity (110).
Alcohols have a sublethal effect on skin microflora and allow very slow bacterial regrowth (25,110). Table 12.11 summarizes the data demonstrating the efficiency of short-chain alcohols for reducing bacteria from artificially contaminated hands at various concentrations and exposure times in a hygienic handwash study (110). The data indicate that alcohols are quickly able to reduce bacteria from hands by 2.6 to 5.8 log10, depending on the type of alcohol and the concentration. Concentration and exposure time are critical for both immediate and residual activity. The greatest residual activity is noted when 2-propanol is combined with chlorhexidine.
Health care workers are less likely to transfer gram-negative bacilli to urinary catheters when they use 2-propanol as a handrub rather than washing with soap (135). The data for rhinoviruses is less striking. Seventy percent ethanol impregnated into towels was only slightly more effective than 10% ethanol. Ethanol-2-propanol and 3% benzyl alcohol were only slightly better than 70% ethanol alone (136). Single treatment with an ethanol handwash was ineffective against rhinovirus (137); however, soap and water was effective. Against influenza H1N1, killing was achieved with up to 70% alcohol-based handrubs, with or without chlorhexidine, although soap and water hand hygiene was slightly more effective (138).
ALDEHYDES
Glutaraldehyde and ortho-Phthalaldehyde
Formaldehyde and glutaraldehyde have been used for sterilization and disinfection of medical devices since the 1960s (139). Although formaldehyde is an excellent biocide, its toxicity has limited its use. Glutaraldehyde is used for routine disinfection of medical devices, especially flexible fiber-optic endoscopes and heat-sensitive medical devices. It has excellent biocidal activity and materials compatibility. ortho-Phthalaldehyde (OPA) is a high-level disinfectant. It has the same materials’ compatibility characteristics of glutaraldehyde and enhanced antimicrobial properties. The structure of these agents is shown in Table 12.1. Many other aldehydes have antimicrobial activity but have not been developed into commercial products and hence will not be discussed here.
Mechanism of Action
Several aldehydes have antimicrobial properties, but the most widely used are glutaraldehyde and formaldehyde. Benzaldehyde, succinaldehyde, malonaldehyde, glyoxal, and several of the β-unsaturated aldehydes (e.g., cinnamaldehyde) also have antimicrobial activity.
The antimicrobial activity of aldehydes in general is based on the reactivity of the aldehyde group (140) and its ability to undergo alkylation reactions. Reactivity of this group can be changed by other functional groups on the molecule. Aldehydes tend to become hydrated in aqueous solutions and in equilibrium with the free aldehyde form, which is believed to be the active moiety. In the case of glutaraldehyde, the monomeric-free aldehyde molecule is in equilibrium with the cyclic hemiacetal and acetal polymers, together coexisting with smaller quantities of mono- and dihydrates. This equilibrium appears to be temperature sensitive, since at higher temperatures, there is a shift to the more active monomeric form. The effect of pH on aldehydes is well described. The reaction of glutaraldehyde with protein increases as the pH rises from 4 to 9 (141). Glutaraldehyde activity is greater at a basic pH of 9 to 10, possibly because condensation reactions are catalyzed better at an alkaline pH (141).
Amines and sulfhydryl groups react most strongly with aldehydes. Cellular constituents involved most often are both enzymes and structural proteins and nucleic acids. Treatment of Micrococcus lysodeikticus cells with glutaraldehyde prevented the release of selective enzymes from the periplasmic space, indicating interaction of glutaraldehyde with the outer cell surface (142). In poliovirus type 1 and echovirus type 25, glutaraldehyde reacts with lysine residues in the protein capsid (143). Enzymatic function via DNA polymerase was affected when the virus was exposed to a glutaraldehyde disinfectant (144). Formaldehyde is known to react with amino groups to bring about intermolecular cross-linking (145) and although it reacts with both RNA and DNA, its reaction with RNA is stronger (146). Glutaraldehyde, like formaldehyde, can cause intramolecular and intermolecular cross-linking of molecules. Antimicrobial properties of OPA involve binding to membrane receptors followed by entrance of the biocide into the cell through the permeable membrane. OPA then compromises cell cycle function through disruption of DNA and RNA (147). Transport of low-molecular-weight amino acids in glutaraldehyde-treated E. coli was reduced about 50%. One would expect much less transport if this was the only mechanism of action (148). In a comparative study of mechanism of action of glutaraldehyde and of OPA on mycobacterial cells, the data suggest that the cross-linking effect of glutaraldehyde on the cell membrane is the mechanism by which glutaraldehyde exerts its antimicrobial action and that the more lipophilic OPA molecule is more efficient at crossing the lipid-rich cell surface of the mycobacterial cell and exerting its action internally (149).
Investigations into the sporicidal activity of glutaraldehyde indicate that the spore coat is protective and that the glutaraldehyde must be alkalinized in order to penetrate this protective barrier (150–152).
The general mechanisms of aldehydes can be summarized as follows:
■ Interaction with cell membrane and cytoplasmic proteins, especially those with sulfhydryl moieties
■ Penetration into cells is pH dependent, with aldehydes working better at basic pH
■ Intramolecular and intermolecular cross-linking of molecules
■ Compromise of cellular function by disrupting DNA and RNA
Spectrum of Activity
Glutaraldehyde was first proposed as an antimicrobial in 1962 (139) as an alternative to formaldehyde sterilization of sutures. The sporicidal properties of alkaline glutaraldehyde were demonstrated in 1963 (153), making this form preferable as a chemical sterilant. OPA is a commercially available high-level disinfectant. Since the only aldehydes commercially available for hospital use in the United States are glutaraldehyde and OPA, the spectrum of activity of other aldehydes, although of interest, is not dealt with here.
The literature describes the effectiveness of glutaraldehyde against gram-positive and gram-negative bacteria. Rubo et al. (154) demonstrated the rapid bactericidal activity of 0.02% glutaraldehyde against Staphylococcus aureus, E. coli, and Pseudomonas aeruginosa. In a 20-minute exposure to this low concentration, inactivation of 104 cells or more was observed. Pseudomonas aeruginosa appeared to be the most resistant of the three organisms tested. Additional work by Borick (155) demonstrated rapid kill (less than 1 minute) of E. coli, Pseudomonas aeruginosa, Serratia marcescens, Proteus vulgaris, and K. pneumonia by 2% glutaraldehyde. Helicobacter pylori was rapidly killed by 0.5% glutaraldehyde with exposure as short as 15 seconds (156). OPA has been shown to be effective against both vancomycin-resistant enterococci (VRE) and MRSA (157).
Several strains of atypical mycobacteria (158–162) were assessed for susceptibility to glutaraldehyde. In evaluating a modified in-use method, it was shown that exposure of strains of Mycobacterium avium-intracellulare, Mycobacterium gordonae, Mycobacterium fortuitum, and M. chelonae to 1% glutaraldehyde for 15 minutes resulted in a 4 log10 reduction, and exposure to a 2% solution for 1 minute resulted in 100% kill (160). In another study, 2% glutaraldehyde tested against several of the same species in a quantitative suspension and carrier test was shown to reduce their populations 5 log10 within 30 minutes in the presence of organic soil and hard water (161). Further work by the same group showed that endoscopes artificially contaminated with M. tuberculosis and M. avium-intracellulare in sputum and endoscopes precleaned using a neutral soap could be disinfected with 2% glutaraldehyde in 10 and 20 minutes, respectively (162). The data indicate that there is a range of times for the inactivation of the various species. Collins (160) found that the most resistant species to glutaraldehyde were M. gordonae and members of the M. avium-intracellulare group (Table 12.12).
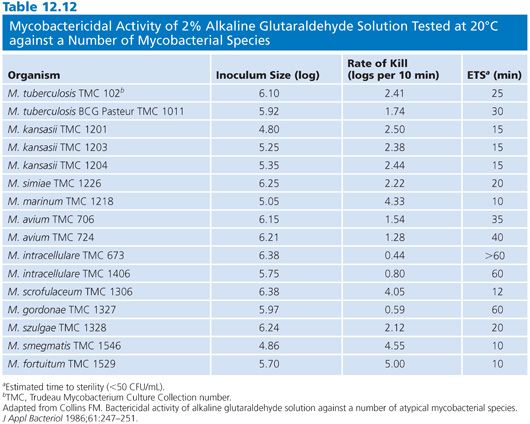
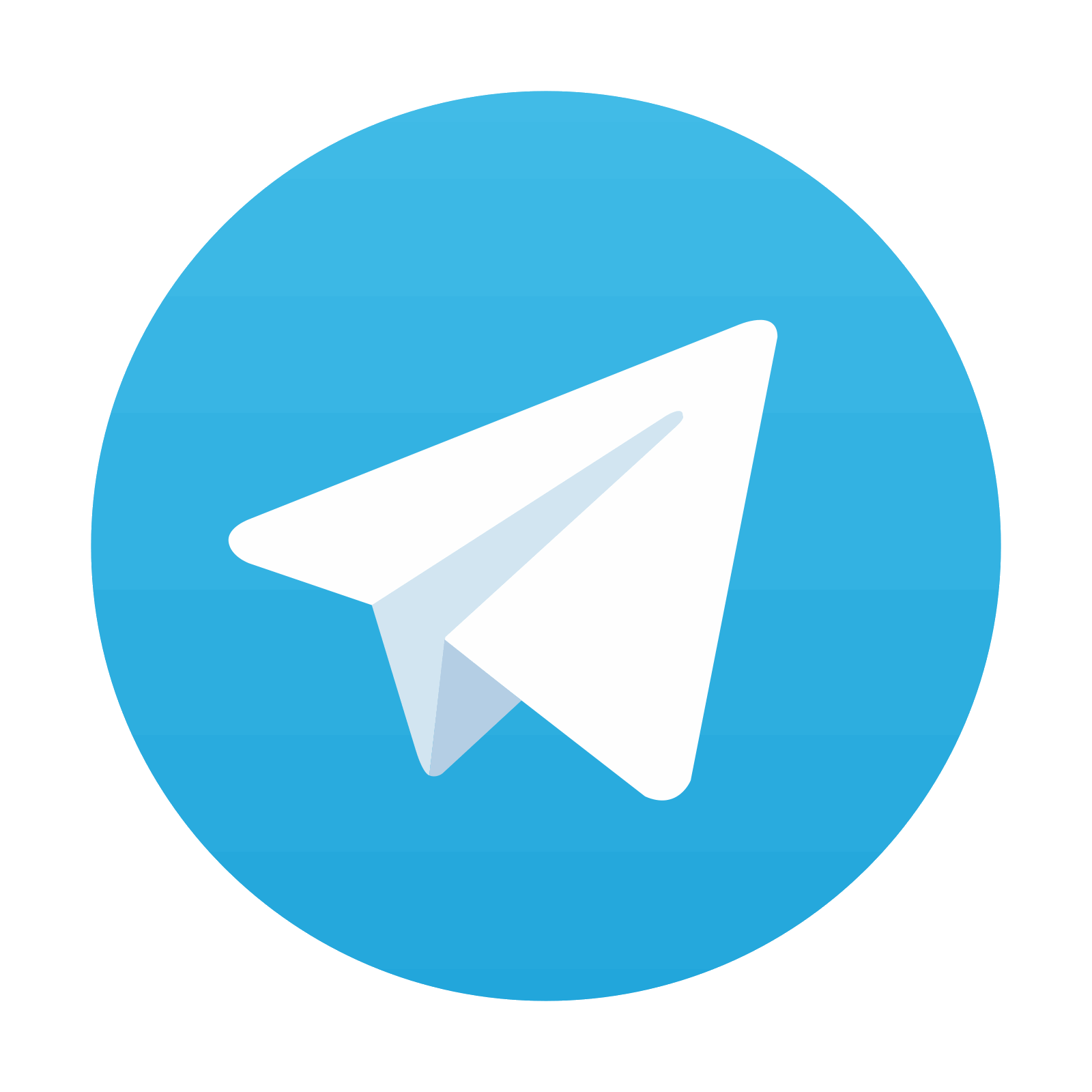
Stay updated, free articles. Join our Telegram channel
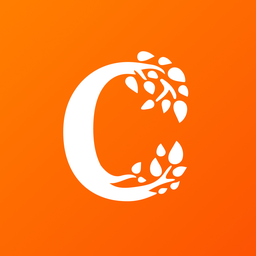
Full access? Get Clinical Tree
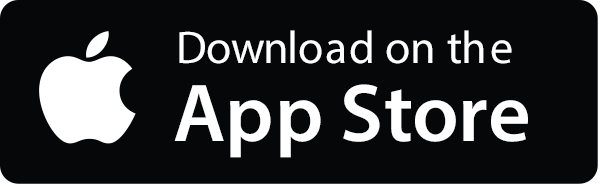
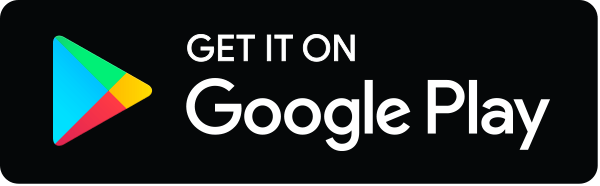