Cholesterol is one of the most well-recognized molecules in human biology, in part because of the direct relationship between its concentrations in blood and tissues and the development of atherosclerotic vascular disease. Cholesterol, which is transported in the blood in lipoprotein complexes because of its absolute insolubility in water, serves as a stabilizing component of cell membranes and is a synthetic precursor of the bile salts and steroid hormones, which participate in cellular signaling. Precursors of cholesterol are converted to ubiquinone, dolichol, and, in the skin, to cholecalciferol, the active form of vitamin D. As a major component of blood lipoproteins, cholesterol can appear in its free, unesterified form in the outer shell of these macromolecules and as cholesterol esters in the lipoprotein core.
Cholesterol is obtained from the diet or synthesized by a pathway that occurs in the cytoplasm of most cells of the body, but to a greater extent in cells of the liver, intestine, and the adrenal cortex and gonads, where cholesterol is used as a precursor for steroid hormone synthesis. The precursor for cholesterol synthesis is acetyl coenzyme A (acetyl CoA), which can be produced from glucose, fatty acids, or amino acids. Two molecules of acetyl CoA form acetoacetyl CoA, which condenses with another molecule of acetyl CoA to form hydroxymethylglutaryl CoA (HMG-CoA). Reduction of HMG-CoA produces mevalonate. This reaction, catalyzed by HMG-CoA reductase, is the major rate-limiting step of cholesterol synthesis. Mevalonate is used to produce isoprene units that condense, eventually forming squalene. Cyclization of squalene produces the steroid ring system, and a number of subsequent reactions generate cholesterol.
Cholesterol is packaged in chylomicrons in the intestine and in very-low-density lipoprotein (VLDL) in the liver. Along with triacylglycerol, cholesterol is transported in the blood in these lipoprotein particles, which also transport triacylglycerols. As the triacylglycerols of the blood lipoproteins are digested by lipoprotein lipase, chylomicrons are converted to chylomicron remnants, and VLDL is converted to intermediate-density lipoprotein (IDL) and subsequently to low-density lipoprotein (LDL). These products return to the liver, where they bind to receptors in cell membranes and are taken up by endocytosis and digested by lysosomal enzymes. LDL is also endocytosed by nonhepatic (peripheral) tissues. Cholesterol and other products of lysosomal digestion are released into the cellular pools. The liver uses this recycled cholesterol, and the cholesterol that is synthesized from acetyl CoA, to produce VLDL and to synthesize bile salts.
Intracellular cholesterol obtained from blood lipoproteins decreases the synthesis of cholesterol within cells, stimulates the storage of cholesterol as cholesterol esters, and decreases the synthesis of LDL receptors. LDL receptors are found on the surface of the cells and bind various classes of lipoproteins before endocytosis.
Although high-density lipoprotein (HDL) contains triacylglycerols and cholesterol, its function is very different from that of the chylomicrons and VLDL, which transport triacylglycerols. HDL exchanges proteins and lipids with the other lipoproteins in the blood. HDL transfers apolipoprotein E (apoE) and apolipoprotein CII (apoCII) to chylomicrons and VLDL. After digestion of the VLDL triacylglycerols in chylomicrons and VLDL, apoE and apoCII are transferred back to HDL. In addition, HDL obtains cholesterol from other lipoproteins and from cell membranes and converts it to cholesterol esters by the lecithin:cholesterol acyltransferase (LCAT) reaction. Then, HDL either directly transports cholesterol and cholesterol esters to the liver or transfers cholesterol esters to other lipoproteins via the cholesterol ester transfer protein (CETP). Ultimately, lipoprotein particles carry the cholesterol and cholesterol esters to the liver, where endocytosis and lysosomal digestion occur. Thus, reverse cholesterol transport (i.e., the return of cholesterol to the liver) is a major function of HDL.
Elevated levels of cholesterol in the blood are associated with the formation of atherosclerotic plaques that can occlude blood vessels, causing heart attacks and strokes. Although high levels of LDL cholesterol are especially atherogenic, high levels of HDL cholesterol are protective because HDL particles are involved in the process of removing cholesterol from tissues, such as the cells lining the blood vessels, and returning it to the liver.
Bile salts, which are produced in the liver from cholesterol obtained from the blood lipoproteins or synthesized from acetyl CoA, are secreted into the bile. They are stored in the gallbladder and released into the intestine during a meal. The bile salts emulsify dietary triacylglycerols, thus aiding in digestion. The digestive products are absorbed by intestinal epithelial cells from bile salt micelles, tiny microdroplets that contain bile salts at their water interface. After the contents of the micelles are absorbed, most of the bile salts travel to the ileum, where they are resorbed and recycled by the liver. Less than 5% of the bile salts that enter the lumen of the small intestine are eventually excreted in the feces.
Although the fecal excretion of bile salts is relatively low, it is a major means by which the body disposes of the steroid nucleus of cholesterol. Because the ring structure of cholesterol cannot be degraded in the body, it is excreted mainly in the bile as free cholesterol and bile salts.
The steroid hormones, derived from cholesterol, include the adrenal cortical hormones (e.g., cortisol, aldosterone, and the adrenal sex steroids dehydroepiandrosterone [DHEA] and androstenedione) and the gonadal hormones (e.g., the ovarian and testicular sex steroids, such as testosterone and estrogen).
THE WAITING ROOM 
At his next office visit, Ivan A.’s case was reviewed by his physician. Ivan A. has several of the major risk factors for coronary heart disease (CHD). These include a sedentary lifestyle, marked obesity, hypertension, hyperlipidemia, and early type 2 diabetes. Unfortunately, he has not been able to follow his doctor’s advice with regard to a diabetic diet designed to effect a significant loss of weight, nor has he followed an aerobic exercise program. As a consequence, his weight has increased from 270 to 291 lb. After a 14-hour fast, his serum glucose is now 214 mg/dL (normal, <100 mg/dL), and his serum total cholesterol level is 314 mg/dL (according to recent guidelines, the LDL cholesterol value has an upper limit, but not total cholesterol). His serum triacylglycerol level is 295 mg/dL (desired level is 150 mg/dL or less), and his serum HDL cholesterol is 24 mg/dL (desired level is ≥40 mg/dL for a man). His calculated serum LDL cholesterol level is 231 mg/dL (when LDL cholesterol is >190 mg/dL treatment is strongly recommended to help prevent cardiovascular disease).
Anne J. was carefully followed by her physician after she survived her heart attack. Before she was discharged from the hospital, she had blood drawn for a fasting lipid panel. After a 14-hour fast, her serum triacylglycerol level was 158 mg/dL (slightly above the upper range of normal), and her HDL cholesterol level was low at 32 mg/dL (normal for women is ≥50 mg/dL). Her serum total cholesterol level was elevated at 420 mg/dL. From these values, her LDL cholesterol level was calculated to be 356 mg/dL (Table 32.1).
TABLE 32.1 Patient Treatment Algorithms
PATIENT STATUS (LINKING AGE WITH LDL CHOLESTEROL LEVELS AND STATUS OF CARDIOVASCULAR DISEASE) AND TREATMENT OPTIONS | TREATMENT RECOMMENDATIONS |
Patient age <75 years with clinical ASCVDa (atherosclerotic cardiovascular disease) |
|
Patient age >75 years of age with clinical ASCVD |
|
Patient age 20–75 years LDL-C level of 190 mg/dL |
|
Patient age 30–75 years of age with heterozygous FH and with an LDL-C level of 100 mg/dL or higher | If maximally tolerated statin therapy and ezetimibe are used, the addition of a PCSK9 inhibitor may be considered. |
Patient age 40–75 years of age with a baseline LDL-C level of 220 mg/dL or higher and who achieve an on-treatment LDL-C level of 130 mg/dL or higher with maximally tolerated statin and ezetimibe treatment | The addition of a PCSK9 inhibitor may be considered. |
Adults 40–75 years of age with diabetes mellitus, regardless of estimated 10-year ASCVD risk | Moderate-intensity statin therapy is indicated. |
Adults 40–75 years of age with LDL levels 70–189 mg/dL | In intermediate-risk patients, LDL-C levels should be reduced by 30% or more, and for optimal ASCVD risk reduction, especially in high-risk patients, by 50% or more. |
aClinical disease refers to acute coronary syndrome, or a history of heart attacks, stable or unstable angina, coronary or other arterial revascularization, stroke, transient ischemic attacks (TIA), or peripheral arterial disease
bA high-intensity statin is a daily dose of statin that lowers LDL cholesterol by approximately ≥50%.
cA moderate-intensity statin is a daily dose of statin that lowers LDL cholesterol by approximately 30% to 50%.
dThe effects of PCSK9 inhibitors and ezetimibe will be described later in the chapter. They are both used for cholesterol-lowering purposes, and work through different mechanisms, and are different from the statin-induced reduction of cholesterol levels.
Data from Grundy SM, Stone HJ, Bailey AL, et al. 2018 AHA/ACC/AACVPR/AAPA/ABC/ACPM/ADA/AGS/APhA/ASPC/NLA/PCNA Guideline on the management of blood cholesterol: a report of the American College of Cardiology/American Heart Association Task Force on Clinical Practice Guidelines. Circulation. 2019;139:e1082–e1143.
Both of Anne J.’s younger brothers had “very high” serum cholesterol levels, and both had suffered heart attacks in their mid-forties. With this information, a tentative diagnosis of familial hypercholesterolemia, type IIA, was made, and the patient was started on a diet and medication as recommended by the American College of Cardiology and the American Heart Association Task Force (see book’s online references). This task force recommends that decisions with regard to when dietary and drug therapy should be initiated is based on the risk of cardiovascular disease (Table 32.2).
TABLE 32.2 Dietary Therapy for Elevated Blood Cholesterol
NUTRIENTa | TARGET |
Saturated Fat | 5%–6% total calories |
Trans fat | Avoid |
Calories | To achieve and maintain desirable body weight |
aAll values are percentage of total calories eaten.
Data from Stone NJ, Robinson JG, Lichtenstein AH, et al. 2013 ACC/AHA Guideline on the treatment of blood cholesterol to reduce atherosclerotic cardiovascular risk in adults: a report of the American College of Cardiology/American Heart Association Task Force on Practice Guidelines. J Am Coll Cardiol. 2014;63:2889–2934.
Anne J. was started on a high-intensity statin, atorvastatin, as she had already experienced a myocardial infarction.
Vera L. is a 32-year-old woman in whom pubertal changes began at age 12, leading to the development of age-appropriate secondary sexual characteristics and the onset of menses at age 13. Her menstrual periods occurred on a monthly basis over the next 7 years, but the flow was scant. At age 20, she noted a gradual increase in her intermenstrual interval from her normal of 28 days to 32 to 38 days. The volume of her menstrual flow also gradually diminished. After 7 months, her menstrual periods ceased. She complained of increasing oiliness of her skin, the appearance of acne-like lesions on her face and upper back, and the appearance of short dark terminal hairs on the mustache and sideburn areas of her face. The amount of extremity hair also increased, and she noticed a disturbing loss of hair from her scalp.
I. Intestinal Absorption of Cholesterol
Cholesterol absorption by intestinal cells is a key regulatory point in human sterol metabolism because it ultimately determines what percentage of the 1,000 mg of biliary cholesterol produced by the liver each day and what percentage of the 300 mg of dietary cholesterol entering the gut per day is eventually absorbed into the blood. In normal subjects, approximately 55% of this intestinal pool enters the blood through the enterocyte each day. The details of cholesterol absorption from dietary sources were outlined in Chapter 29.
Although the absorption of cholesterol from the intestinal lumen is a diffusion-controlled process, there is also a mechanism to remove unwanted or excessive cholesterol and plant sterols from the enterocyte. The transport of sterols out of the enterocyte and into the gut lumen is related to the products of genes that code for the adenosine triphosphate (ATP)-binding cassette (ABC) transporter protein family, specifically ABCG5 and ABCG8. These proteins form a complex called sterolin that couples ATP hydrolysis to the transport of unwanted or excessive cholesterol and plant sterols (phytosterols) from the enterocyte back into the gut lumen. Another member of the ABC family, ABCA1, is required for reverse cholesterol transport and the biogenesis of HDL. Cholesterol cannot be metabolized to CO2 and water and is, therefore, eliminated from the body principally in the feces as unreabsorbed sterols and bile acids. ABC transporter protein expression increases the amount of sterols present in the gut lumen, with the potential to increase the elimination of the sterols into the feces. Patients with a condition known as sitosterolemia (a rare autosomal recessive disease, also known as phytosterolemia) have a defect in the function of either ABCG5 or ABCG8 in the enterocytes, which leads to the accumulation of cholesterol and phytosterols within these cells. These eventually reach the bloodstream, markedly elevating the level of cholesterol and phytosterol in the blood. This accounts for the increased cardiovascular morbidity in individuals with this disorder. From these genetic anomalies, it is clear that agents that either amplify the expression of the ABC transporter proteins within enterocytes or that block cholesterol absorption from the lumen have therapeutic potential in the treatment of patients with hypercholesterolemia. Ezetimibe is a compound that, although structurally distinct from the sterols, lowers serum cholesterol levels by blocking cholesterol absorption by the enterocyte. The target of ezetimibe is the Niemann–Pick C1-like 1 protein (NPC1L1), which is believed to transport cholesterol into cells via an absorptive endocytotic mechanism, involving the protein clathrin coat protein. The reduction of cholesterol absorption from the intestinal lumen has been shown to reduce blood levels of LDL cholesterol, particularly when used with a drug which also blocks endogenous cholesterol synthesis.
II. Cholesterol Synthesis
Cholesterol is an alicyclic compound whose basic structure contains four fused rings (Fig. 32.1). In its “free” form, the cholesterol molecule contains a number of modifications to the basic ring structure (see Fig. 32.1B). Of note is the hydroxyl group at C3 of the A ring. Approximately one-third of plasma cholesterol exists in the free (or unesterified) form. The remaining two-thirds exist as cholesterol esters in which a long-chain fatty acid (usually linoleic acid) is attached by ester linkage to the hydroxyl group at C3 of the A ring. Esterified cholesterol is more hydrophobic than free cholesterol due to this modification. The proportions of free and esterified cholesterol in the blood can be measured using methods such as high-performance liquid chromatography (HPLC).
FIGURE 32.1 The steroid ring nucleus and cholesterol. A. The basic ring structure of sterols; the perhydrocyclopentanophenanthrene nucleus. Each ring is labeled A, B, C, or D. B. The structure of cholesterol.
The synthesis of cholesterol, like that of fatty acids, occurs in the cytoplasm and requires significant reducing power, which is supplied in the form of NADPH. The NADPH is produced by the HMP shunt pathway. All 27 carbons are derived from one precursor, acetyl CoA. Acetyl CoA can be obtained from several sources, including the β-oxidation of fatty acids, the oxidation of ketogenic amino acids, such as leucine and lysine, and the pyruvate dehydrogenase reaction. Energy is also required, which is provided by the hydrolysis of high-energy thioester bonds of acetyl CoA and phosphoanhydride bonds of ATP. Cholesterol synthesis occurs in four stages.
A. Stage 1: Synthesis of Mevalonate from Acetyl CoA
The first stage of cholesterol synthesis leads to the production of the intermediate mevalonate (Fig. 32.2). The synthesis of mevalonate is the committed, rate-limiting step in cholesterol formation. In this cytoplasmic pathway, two molecules of acetyl CoA condense, forming acetoacetyl CoA, which then condenses with a third molecule of acetyl CoA to yield the 6-carbon compound 3-hydroxy-methylglutaryl-CoA (HMG-CoA). The HMG-CoA synthase in this reaction is present in the cytosol and is distinct from the mitochondrial HMG-CoA synthase that catalyzes HMG-CoA synthesis involved in the production of ketone bodies. The committed step and major point of regulation of cholesterol synthesis in stage 1 involves reduction of HMG-CoA to mevalonate, a reaction that is catalyzed by HMG-CoA reductase, an enzyme embedded in the membrane of the endoplasmic reticulum. HMG-CoA reductase contains eight membrane-spanning domains, and the carboxy-terminal domain, which faces the cytoplasm, possesses the enzymatic activity. The reducing equivalents for this reaction are donated by two molecules of NADPH. The regulation of the activity of HMG-CoA reductase is controlled in multiple ways and is described in Section II.E.
FIGURE 32.2 The conversion of three molecules of acetyl coenzyme A (acetyl CoA) to mevalonic acid. acetoacetyl CoA, acetoacetyl coenzyme A; CoA, coenzyme A; HMG-CoA, 3-hydroxymethylglutaryl coenzyme A; NADP, nicotinamide adenine dinucleotide phosphate.
B. Stage 2: Conversion of Mevalonate to Two Activated Isoprenes
In the second stage of cholesterol synthesis, three phosphate groups are transferred from three molecules of ATP to mevalonate (Fig. 32.3). The purpose of these phosphate transfers is to activate both carbon 5 and the hydroxyl group on carbon 3 for further reactions in which these groups will participate. The phosphate group attached to the C3 hydroxyl group of mevalonate in the 3-phospho-5-pyrophosphomevalonate intermediate is removed along with the carboxyl group on C1. This produces a double bond in the 5-carbon product, Δ3-isopentenyl pyrophosphate, the first of two activated isoprenes that are necessary for the synthesis of cholesterol. The second activated isoprene is formed when Δ3-isopentenyl pyrophosphate is isomerized to dimethylallyl pyrophosphate (see Fig. 32.3). Isoprenes, in addition to being used for cholesterol biosynthesis, are also utilized in the synthesis of coenzyme Q and dolichol.
FIGURE 32.3 The formation of activated isoprene units (Δ3-isopentenyl pyrophosphate and dimethylallyl pyrophosphate) from mevalonic acid. Note the large adenosine triphosphate (ATP) requirement for these steps. ADP, adenosine diphosphate; Pi, inorganic phosphate.
C. Stage 3: Condensation of Six Activated 5-Carbon Isoprenes to Squalene
The next stage in the biosynthesis of cholesterol involves the head-to-tail condensation of isopentenyl pyrophosphate and dimethylallyl pyrophosphate. The “head” in this case refers to the end of the molecule to which pyrophosphate is linked. In this reaction, the pyrophosphate group of dimethylallyl pyrophosphate is displaced, and a 10-carbon chain, known as geranyl pyrophosphate, is generated (Fig. 32.4). Geranyl pyrophosphate then undergoes another head-to-tail condensation with isopentenyl pyrophosphate, resulting in the formation of the 15-carbon intermediate, farnesyl pyrophosphate. After this, two molecules of farnesyl pyrophosphate undergo a head-to-head fusion, and both pyrophosphate groups are removed to form squalene, a compound that was first isolated from the liver of sharks (genus Squalus). Squalene contains 30 carbons (24 in the main chain and 6 in the methyl group branches; see Fig. 32.4).
FIGURE 32.4 The formation of squalene from six isoprene units. The activation of the isoprene units drives their condensation to form geranyl pyrophosphate, farnesyl pyrophosphate, and squalene. NADP, nicotinamide adenine dinucleotide phosphate; PPi, inorganic pyrophosphate.
D. Stage 4: Conversion of Squalene to the Steroid Nucleus
The enzyme squalene monooxygenase adds a single oxygen atom from O2 to the end of the squalene molecule, forming an epoxide. NADPH then reduces the other oxygen atom of O2 to H2O. The unsaturated carbons of the squalene 2,3-epoxide are aligned in a way that allows conversion of the linear squalene epoxide into a cyclic structure. The cyclization leads to the formation of lanosterol, a sterol with the four-ring structure characteristic of the steroid nucleus. A series of complex reactions, containing many steps and elucidated in the late 1950s, leads to the formation of cholesterol (Fig. 32.5).
FIGURE 32.5 The conversion of squalene to cholesterol. Squalene is shown in a different conformation than in Figure 32.4 to indicate better how the cyclization reaction occurs. NADP, nicotinamide dinucleotide phosphate.
E. Regulation of HMG-CoA Reductase
HMG-CoA reductase is the rate-limiting step of cholesterol biosynthesis and, as such, is highly regulated. It is also the target of the statins, which are cholesterol-lowering drugs. The regulation of HMG-CoA reductase activity is controlled in multiple ways, including transcriptional regulation, regulation of the amount of enzyme by proteolysis, and covalent modification.
1. Transcriptional Regulation
The rate of synthesis of HMG-CoA reductase messenger RNA (mRNA) is controlled by one of the family of sterol-regulatory element-binding proteins (SREBPs) (Fig. 32.6A). These transcription factors belong to the helix–loop–helix–leucine zipper (bHlH-Zip) family of transcription factors that directly activate the expression of >30 genes dedicated to the synthesis and uptake of cholesterol, fatty acids, triacylglycerols, and phospholipids as well as the production of the NADPH cofactors required to synthesize these molecules. A simplified view of the process is described in the following paragraph.
FIGURE 32.6 Regulation of 3-hydroxymethylglutaryl coenzyme A (HMG-CoA) reductase activity. See text for details and abbreviations. A. Transcriptional control. B. Regulation by proteolysis. C. Regulation by phosphorylation. ADP, adenosine diphosphate; AMP, adenosine monophosphate; ATP, adenosine triphosphate; ER, endoplasmic reticulum; Pi, inorganic phosphate; SCAP, SREBP cleavage-activating protein; SRE, sterol-regulatory element; SREBP, sterol-regulatory element-binding proteins; SIP, site I protease; S2P, site 2 protease.
SREBPs specifically enhance transcription of the HMG-CoA reductase gene by binding to the sterol-regulatory element (SRE) upstream of the gene. When bound, the rate of transcription is increased. SREBPs are integral proteins of the endoplasmic reticulum (ER). The SREBP is bound to SCAP (SREBP cleavage-activating protein) in the ER membrane when cholesterol levels are high. When cholesterol levels drop, the sterol leaves its SCAP-binding site, and the SREBP:SCAP complex is transported to the Golgi apparatus in coat protein complex II (COPII) containing vesicles. Within the Golgi, two proteolytic cleavages occur (via the site 1 [S1P] and site 2 [S2P] proteases), which release the N-terminal transcription factor domain from the Golgi membrane. Once released, the active amino-terminal component travels to the nucleus to bind to SREs. The soluble SREBPs are rapidly turned over and need to be continuously produced to stimulate reductase mRNA transcription effectively. When cytoplasmic sterol levels rise, the sterols bind to SCAP and prevent translocation of the complex to the Golgi, leading to a decrease in transcription of the reductase gene and thus less reductase protein being produced.
2. Proteolytic Degradation of HMG-CoA reductase
Rising levels of cholesterol and bile salts in cells that synthesize these molecules also may cause a change in the oligomerization state of the membrane domain of HMG-CoA reductase, rendering the enzyme more susceptible to ubiquitination and proteolysis by the proteasome (see Fig. 32.6B). This, in turn, decreases its activity. The membrane domains of HMG-CoA reductase contain sterol-sensing regions, which are similar to those in SCAP.
3. Regulation by Covalent Modification
In addition to the inductive and repressive pathways previously mentioned, the activity of the reductase is also regulated by phosphorylation and dephosphorylation (see Fig. 32.6C). Elevated glucagon levels increase phosphorylation of the enzyme, thereby inactivating it, whereas hyperinsulinemia increases the activity of the reductase by activating phosphatases, which dephosphorylate the reductase. Increased levels of intracellular sterols may also increase phosphorylation of HMG-CoA reductase, thereby reducing its activity as well (feedback inhibition). Thyroid hormone also increases enzyme activity, whereas glucocorticoids decrease its activity. The enzyme that phosphorylates HMG-CoA reductase is the adenosine monophosphate (AMP)-activated protein kinase, which itself is regulated by phosphorylation by one of several AMP-activated protein kinases (one of which is LKB1, see later). Thus, cholesterol synthesis decreases when ATP levels are low and increases when ATP levels are high, similar to what occurs with fatty acid synthesis (recall that acetyl CoA carboxylase is also phosphorylated and inhibited by the AMP-activated protein kinase). As has been seen during the discussion of cholesterol biosynthesis, large amounts of ATP are required to synthesize cholesterol, so low energy levels will lead to inhibition of the pathway.
III. Several Fates of Cholesterol
Almost all mammalian cells are capable of producing cholesterol. Most of the biosynthesis of cholesterol occurs within liver cells, although the gut, the adrenal cortex, and the gonads (as well as the placenta in pregnant women) also produce significant quantities of the sterol. A fraction of hepatic cholesterol is used for the synthesis of hepatic membranes, but the bulk of synthesized cholesterol is secreted from the hepatocyte as one of three moieties: cholesterol esters, biliary cholesterol (cholesterol found in the bile), or bile acids. Cholesterol ester production in the liver is catalyzed by acyl CoA–cholesterol acyl transferase (ACAT). ACAT catalyzes the transfer of a fatty acid from coenzyme A to the hydroxyl group on carbon 3 of cholesterol (Fig. 32.7). The liver packages some of the very hydrophobic esterified cholesterol into the hollow core of lipoproteins, primarily VLDL. VLDL is secreted from the hepatocyte into the blood and transports the cholesterol esters (and triacylglycerols, phospholipids, apolipoproteins, etc.) to the tissues that require greater amounts of cholesterol than they can synthesize de novo. These tissues then use the cholesterol for the synthesis of membranes, for the formation of steroid hormones, and for the biosynthesis of vitamin D. The residual cholesterol esters not used in these ways are stored in the liver for later use.
FIGURE 32.7 The acyl coenzyme A cholesterol acyltransferase (ACAT) reaction, producing cholesterol esters. CoA, coenzyme A.
The hepatic cholesterol pool also serves as a source of cholesterol for the synthesis of the relatively hydrophilic bile acids and their salts (see Chapter 29). These derivatives of cholesterol are very effective detergents because they contain both polar and nonpolar regions. They are introduced into the biliary ducts of the liver. They are stored and concentrated in the gallbladder and later discharged into the gut in response to the ingestion of food. They aid in the digestion of intraluminal lipids by forming micelles with them, which increases the surface area of lipids exposed to the digestive action of intraluminal lipases. Free cholesterol also enters the gut lumen via the biliary tract (approximately 1,000 mg daily, which mixes with 300 mg of dietary cholesterol to form an intestinal pool, roughly 55% of which is resorbed by the enterocytes and enters the bloodstream daily). On a low-cholesterol diet, the liver synthesizes approximately 800 mg of cholesterol per day to replace bile salts and cholesterol lost from the enterohepatic circulation into the feces. Conversely, a greater intake of dietary cholesterol suppresses the rate of hepatic cholesterol synthesis (feedback inhibition).
IV. Synthesis of Bile Salts
A. Conversion of Cholesterol to Cholic Acid and Chenodeoxycholic Acid
Bile salts are synthesized in the liver from cholesterol by reactions that hydroxylate the steroid nucleus and cleave the side chain. In the first and rate-limiting reaction, an α-hydroxyl group is added to carbon 7 (on the α side of the B-ring). The activity of the 7α-hydroxylase that catalyzes this step is decreased by an increase in bile salt concentration (Fig. 32.8).
FIGURE 32.8 The reaction catalyzed by 7α-hydroxylase. An α-hydroxyl group is formed at position 7 of cholesterol. This reaction, which is inhibited by bile salts, is the rate-limiting step in bile salt synthesis. NADP, nicotinamide adenine dinucleotide phosphate.
In subsequent steps, the double bond in the B-ring is reduced, and an additional hydroxylation may occur. Two different sets of compounds are produced. One set has α-hydroxyl groups at positions 3, 7, and 12 and produces the cholic acid series of bile salts. The other set has α-hydroxyl groups at positions 3 and 7 and produces the chenodeoxycholic acid series (also known as the chenocholic acid series, Fig. 32.9). Three carbons are removed from the side chain by an oxidation reaction. The remaining five-carbon fragment attached to the ring structure contains a carboxyl group (see Fig. 32.9).
FIGURE 32.9 Synthesis of bile salts. Two sets of bile salts are generated; one with α-hydroxyl groups at positions 3 and 7 (the chenodeoxycholate series) and the other with α-hydroxyls at positions 3, 7, and 12 (the cholate series). Note how bile salt accumulation will inhibit the initial step of the pathway, catalyzed by 7α-hydroxylase.
The pKa of the bile acids is approximately 6. Therefore, in the contents of the intestinal lumen, which normally have a pH of 6, approximately 50% of the molecules are present in the protonated form and 50% are ionized, which form bile salts. (The terms bile acids and bile salts are often used interchangeably, but bile salts actually refer to the ionized form of the molecules.)
B. Conjugation of Bile Salts
The carboxyl group at the end of the side chain of the bile salts is activated by a reaction that requires ATP and coenzyme A. The CoA derivatives can react with either glycine or taurine (which is derived from cysteine), forming amides that are known as conjugated bile salts (Fig. 32.10). In glycocholic acid and glycochenodeoxycholic acid, the bile acids are conjugated with glycine. These compounds have a pKa of approximately 4, so compared to their unconjugated forms, a higher percentage of the molecules is present in the ionized form at the pH of the intestine. The taurine conjugates, taurocholic and taurochenodeoxycholic acid, have a pKa of approximately 2. Therefore, compared with the glycoconjugates, an even greater percentage of the molecules of these conjugates is ionized in the lumen of the gut.
FIGURE 32.10 Conjugation of bile salts. Conjugation lowers the pKa of the bile salts, making them better detergents; that is, they are more ionized in the contents of the intestinal lumen (pH ≈ 6) than are the unconjugated bile salts (pKa ≈ 6). The reactions are the same for the chenodeoxycholic acid series of bile salts. ADP, adenosine diphosphate; AMP, adenosine monophosphate; ATP, adenosine triphosphate; CoA, coenzyme A; PPi, pyrophosphate.
C. Fate of the Bile Salts
Bile salts are produced in the liver and secreted into the bile (Fig. 32.11). They are stored in the gallbladder and released into the intestine during a meal, where they serve as detergents that aid in the digestion of dietary lipids (see Chapter 29).
FIGURE 32.11 Overview of bile salt metabolism.
Intestinal bacteria deconjugate and dehydroxylate the bile salts, removing the glycine and taurine residues and the hydroxyl group at position 7. The bile salts that lack a hydroxyl group at position 7 are called secondary bile salts. The deconjugated and dehydroxylated bile salts are less soluble and, therefore, are less readily resorbed from the intestinal lumen than the bile salts that have not been subjected to bacterial action (Fig. 32.12). Lithocholic acid, a secondary bile salt that has a hydroxyl group only at position 3, is the least soluble bile salt. Its major fate is excretion.
FIGURE 32.12 Structures of the primary and secondary bile salts. Primary bile salts form conjugates with taurine or glycine in the liver. After secretion into the intestine, they may be deconjugated and dehydroxylated by the bacterial flora, forming secondary bile salts. Note that dehydroxylation occurs at position 7, forming the deoxy family of bile salts. Dehydroxylation at position 12 also leads to the excretion of the bile salt.
Greater than 95% of the bile salts are resorbed in the ileum and return to the liver via the enterohepatic circulation (via the portal vein; see Fig. 32.11). The secondary bile salts may be reconjugated in the liver, but they are not rehydroxylated. The bile salts are recycled by the liver, which secretes them into the bile. This enterohepatic recirculation of bile salts is extremely efficient. Less than 5% of the bile salts entering the gut are excreted in the feces each day. Because the steroid nucleus cannot be degraded in the body, the excretion of bile salts serves as a major route for removal of the steroid nucleus and thus of cholesterol from the body.
V. Transport of Cholesterol by the Blood Lipoproteins
Because they are hydrophobic and essentially insoluble in the water of the blood, cholesterol and cholesterol esters, like triacylglycerols and phospholipids, must be transported through the bloodstream packaged as lipoproteins. These macromolecules are water soluble. Each lipoprotein particle is composed of a core of hydrophobic lipids such as cholesterol esters and triacylglycerols surrounded by a shell of polar lipids (the phospholipids), and a variety of apolipoproteins, which allows a hydration shell to form around the lipoprotein (see Fig. 29.7). This occurs when the positive charge of the nitrogen atom of the phospholipid (phosphatidylcholine, phosphatidylethanolamine, or phosphatidylserine) forms an ionic bond with the negatively charged hydroxyl ion of the environment. The surface-bound apolipoproteins also increase the water solubility of the lipoprotein particle. Free cholesterol molecules are dispersed throughout the lipoprotein shell to stabilize it in a way that allows it to maintain its spherical shape. The major carriers of lipids are chylomicrons (see Chapter 29), VLDL, and HDL. Metabolism of VLDL leads to IDL and LDL. Metabolism of chylomicrons leads to the formation of chylomicron remnants.
Through this carrier mechanism, lipids leave their tissue of origin, enter the bloodstream, and are transported to the tissues, where their components are either used in synthetic or oxidative process or stored for later use. The apolipoproteins (“apo” describes the protein within the shell of the particle in its lipid-free form) not only add to the hydrophilicity and structural stability of the particle, they have other functions as well: (1) They activate certain enzymes required for normal lipoprotein metabolism and (2) they act as ligands on the surface of the lipoprotein that target-specific receptors on peripheral tissues that require lipoprotein delivery for their innate cellular functions.
Ten principal apolipoproteins have been characterized. Their tissue sources, molecular mass, distribution within lipoproteins, and metabolic functions are shown in Table 32.3.
TABLE 32.3 Characteristics of the Major Apolipoproteins
HDL, high-density lipoprotein; IDL, intermediate-density lipoprotein; LCAT, lecithin-cholesterol acyltransferase; LDL, low-density lipoprotein; VLDL, very-low-density lipoprotein.
Adapted from Feingold KR. Introduction to lipids and lipoproteins. Endotext [Internet]. https://www.ncbi.nlm.nih.gov/books/NBK305896/
The lipoproteins themselves have been assigned to eight major classes. Some of their characteristics are shown in Table 32.4. Each class of lipoprotein has a specific function determined by its apolipoprotein content, its tissue of origin, and the proportion of the macromolecule made up of triacylglycerols, cholesterol esters, free cholesterol, and phospholipids (see Tables 32.3 and 32.4).
TABLE 32.4 Characteristics of the Major Lipoproteins
Chol, the sum of free and esterified cholesterol; IDL, intermediate-density lipoprotein; HDL, high-density lipoprotein; LDL, low-density lipoprotein; PL, phospholipid; TG, triacylglycerols; VLDL, very-low-density lipoprotein.
aThe remaining percent composition is composed of apolipoproteins.
A. Chylomicrons
Chylomicrons are the largest of the lipoproteins and the least dense because of their rich triacylglycerol content. They are assembled from dietary lipids (the exogenous lipoprotein pathway) within the epithelial cells of the small intestine and then secreted into the lymphatic vessels draining the gut (see Fig. 29.10). They enter the bloodstream via the left subclavian vein. The major apolipoproteins of chylomicrons are apolipoprotein B-48 (apoB-48), apoCII, and apoE (see Table 32.3). The apoCII activates lipoprotein lipase (LPL), an enzyme that projects into the lumen of capillaries in adipose tissue, cardiac muscle, skeletal muscle, and the acinar cells of mammary tissue. This activation allows LPL to hydrolyze the triacylglycerol of the chylomicrons, leading to the release of free fatty acids. The muscle cells then oxidize the fatty acids as fuel, while the adipocytes and mammary cells store them as triacylglycerols (fat) or, in the case of the lactating breast, use them for milk formation. The partially hydrolyzed chylomicrons remaining in the bloodstream (the chylomicron remnants), now partly depleted of their core triacylglycerols, have lost their apoCII but still retain their apoE and apoB-48 proteins. Receptors in the plasma membranes of the liver cells bind to apoE on the surface of these remnants, allowing them to be taken up by the liver through a process of receptor-mediated endocytosis (see later).
B. Very-Low-Density Lipoprotein
If dietary intake of carbohydrates exceeds the immediate fuel requirements of the liver, the excess carbohydrates are converted to triacylglycerols, which, along with free and esterified cholesterol, phospholipids, and the major apolipoprotein apoB-100 (see Table 32.3), are packaged to form nascent VLDL. These particles are then secreted from the liver (the “endogenous” pathway of lipoprotein metabolism) into the bloodstream (Fig. 32.13), where they accept apoCII and apoE from circulating HDL particles. This then forms the mature VLDL particle. These particles are then transported from the hepatic veins to capillaries in skeletal and cardiac muscle and adipose tissue, as well as lactating mammary tissues, where lipoprotein lipase is activated by apoCII in the VLDL particles. The activated enzyme facilitates the hydrolysis of the triacylglycerol in VLDL, causing the release of fatty acids and glycerol from a portion of core triacylglycerols. These fatty acids are oxidized as fuel by muscle cells, used in the resynthesis of triacylglycerols in fat cells, and used for milk production in the lactating breast. As the VLDL particles are depleted of triacylglycerol, VLDL remnants are formed. Approximately 50% of these remnants are taken up from the blood by liver cells through the binding of VLDL apoE to the hepatocyte plasma membrane apoE receptor, followed by endocytic internalization of the VLDL remnant (similar to the fate of the chylomicron remnant).
FIGURE 32.13 Fate of very-low-density lipoprotein (VLDL). VLDL triacylglycerol (TG) is degraded by lipoprotein lipase (LPL), forming intermediate-density lipoprotein (IDL). IDL can either be endocytosed by the liver through a receptor-mediated process or further digested, mainly by hepatic triacylglycerol lipase (HTGL), to form low-density lipoprotein (LDL). LDL may be endocytosed by receptor-mediated processes in the liver or in peripheral cells. LDL also may be oxidized and taken up by “scavenger” receptors on macrophages. The scavenger pathway plays a role in atherosclerosis. FA, fatty acids; Pi, inorganic phosphate.
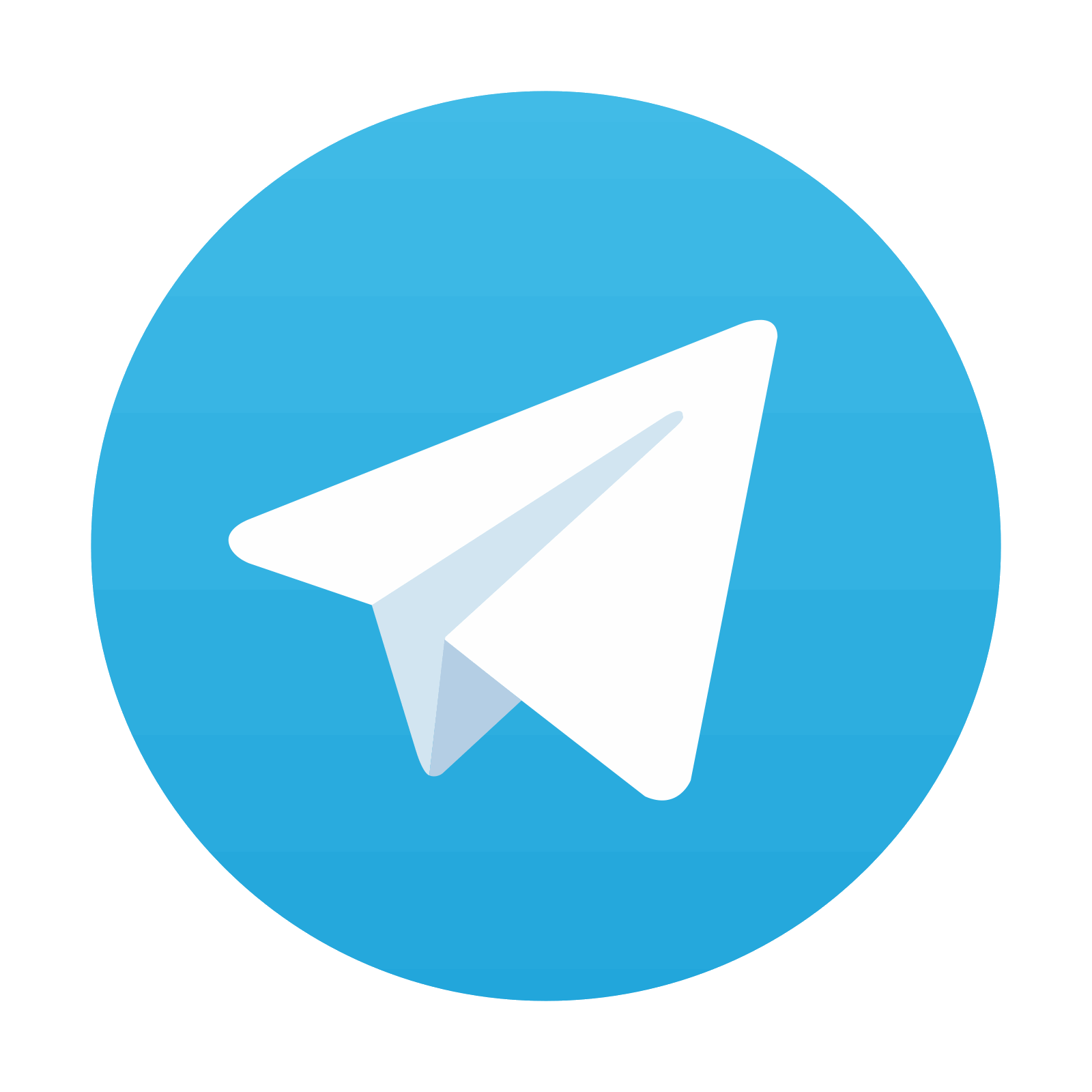
Stay updated, free articles. Join our Telegram channel
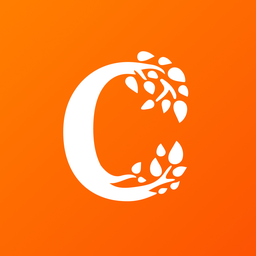
Full access? Get Clinical Tree
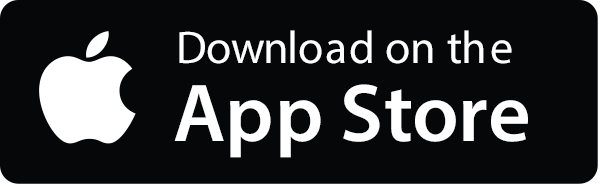
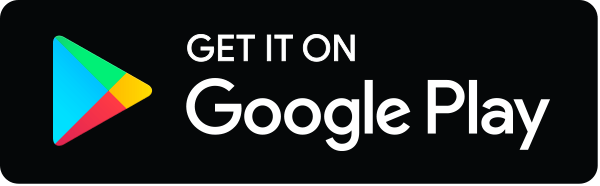