Chapter 30 Graeme Eisenhofer, Ph.D., Ronald J. Whitley, Ph.D., D.A.B.C.C. and Thomas G. Rosano, Ph.D., D.A.B.C.C, D.A.B.F.T. The catecholamines produced naturally in humans—including dopamine, norepinephrine (noradrenaline), and epinephrine (adrenaline)—are phenylethylamines with hydroxyl groups on positions three and four of the benzene ring and an ethylamine sidechain on position one (Figure 30-1). Hydroxyl and methyl substitutions on the ethylamine sidechain distinguish the individual catecholamines in both structure and function. The catecholamines demonstrate varying degrees of alkaline instability in biological fluids, and their dihydroxybenzene or catechol structure is sensitive to oxidative formation of quinones in the presence of air and light. Several pharmaceutical and designer drugs with the basic phenylethylamine structure also have effects on related neuroeffector systems; such drugs are commonly referred to as sympathomimetic agents. Serotonin with its indoleamine structure is distinct from the catecholamines (see Figure 30-1), and is an important naturally occurring biogenic amine produced widely in animal and plant kingdoms. Serotonin is structurally related to melatonin, the principle indoleamine produced by the pineal gland. A number of natural or synthetic congeners of serotonin have biological effects ranging from cerebral vasoconstriction (e.g., sumatripan) to psychoactive hallucinogenic actions (e.g., N,N-dimethyltryptamine). Catecholamines are synthesized from the amino acid, tyrosine, and serotonin from tryptophan (Figure 30-2). The rate-limiting step in catecholamine biosynthesis involves conversion of tyrosine to 3,4-dihydroxyphenylalanine (L-dopa) by the enzyme, tyrosine hydroxylase.191 A related enzyme, tryptophan hydroxylase, catalyzes conversion of tryptophan to 5-hydroxytryptophan in the first step of serotonin synthesis. In contrast to tyrosine hydroxylase, which is encoded by one gene, tryptophan hydroxylase is encoded by two genes and exists as two distinct isoforms; one of these isoforms is expressed in the central nervous system and the other at peripheral sites of serotonin synthesis, including the gut, pineal gland, spleen, and thymus.258 Tissue sources of catecholamines are principally dependent on the presence of tyrosine hydroxylase, which is largely confined to dopaminergic and noradrenergic neurons of the central nervous system, and to sympathetic nerves and chromaffin cells of the adrenal medulla and paraganglia in the periphery. Similarly, sources of serotonin are largely dependent on the presence of tryptophan hydroxylase in central nervous system serotonergic neurons, the pineal gland, and some peripheral endocrine tissues, particularly enterochromaffin cells of the digestive tract. Platelets also contain large amounts of serotonin; however, this is derived from serotonin synthesized in enterochromaffin cells of the gastrointestinal tract.242 Both tyrosine and tryptophan hydroxylases belong to a small family of mono-oxygenases that also includes phenylalanine hydroxylase; all three enzymes require tetrahydrobiopterin as a substrate to drive the hydroxylation reaction.79 Deficiencies in the enzymes responsible for formation and recycling of tetrahydrobiopterin result in variant forms of phenylketonuria and hyperphenylalaninemia characterized by low concentrations of monoamine neurotransmitters and severe neurologic abnormalities.130 Conversion of L-dopa to dopamine and of 5-hydroxytryptophan to serotonin is catalyzed by aromatic-L-amino acid decarboxylase (see Figure 30-2), an enzyme with a wide tissue distribution and broad substrate specificity for aromatic amino acids and requiring pyridoxal-5-phosphate as a cofactor. The dopamine and serotonin formed in the cytoplasm are then transported into vesicular storage granules, where the amines are available for exocytotic release as the principal neurotransmitters of central nervous system dopaminergic and serotonergic neurons. The dopamine formed in noradrenergic neurons and chromaffin cells is further converted to norepinephrine by dopamine β-hydroxylase, a copper-containing enzyme requiring molecular oxygen and ascorbic acid for activity. The enzyme has a unique presence in vesicular storage granules, bound to the vesicular membrane or present in the soluble matrix core. The noradrenergic neurochemical phenotype of central noradrenergic neurons and peripheral sympathetic nerves depends on both translocation of dopamine into storage granules and the presence of dopamine β-hydroxylase. The additional presence of phenylethanolamine N-methyltransferase (PNMT) in adrenal medullary chromaffin cells leads to further conversion of norepinephrine to epinephrine (see Figure 30-2). Because PNMT is a cytosolic enzyme, this step depends on leakage of norepinephrine from vesicular storage granules into the cell cytoplasm, where the amine is available for N-methylation. Epinephrine is then translocated into chromaffin granules, where it is stored awaiting release. Melatonin is synthesized from serotonin in the pineal gland by two highly specific enzymes, the first step catalyzed by serotonin-N-acetyltransferase and the second by hydroxyindole-O-methyltransferase (see Figure 30-2). Synthesis of melatonin in the pineal gland is regulated by the 24-hour light/dark cycle, resulting in pronounced diurnal fluctuations in production, with higher melatonin concentrations at night associated with induction of sleep. Conversion of tyrosine to L-dopa by tyrosine hydroxylase and conversion of tryptophan to 5-hydroxytrytophan by tryptophan hydroxylase represent pivotal points for regulating synthesis and maintaining stores of catecholamines and serotonin in response to changes in monoamine turnover associated with variations in exocytotic release. Rapid activation of tyrosine hydroxylase is achieved by phosphorylation of serine residues at the regulatory domain, under the control of multiple Ca2+ and cyclic adenosine monophosphate (cAMP)-dependent pathways influenced by changes in nerve activity and actions of peptides and other coactivators.192,275 Feedback inhibition by catecholamines provides a further mechanism for short-term regulation of enzyme activity. Long-term regulation involves induction of synthesis of the enzyme at the transcriptional level. Similar mechanisms are involved in the regulation of tryptophan hydroxylase, although these differ for the two isoforms expressed at central nervous system and peripheral sites of serotonin synthesis.190 Synthesis of serotonin is also controlled by the availability of tryptophan precursor derived exclusively from the diet.271 Tyrosine, in contrast, is additionally derived by hydroxylation of phenylalanine. Storage of catecholamines and serotonin in vesicular granules is facilitated by two vesicular monoamine transporters.108 Both transporters have a wide specificity for different monoamine substrates. The driving force for vesicular monoamine transport is provided by an adenosine triphosphate–dependent vesicular membrane proton pump, which maintains an H+ electrochemical gradient between the cytoplasm and granule matrix.230 Disruption of this gradient in situations of energy depletion and lowered intracellular pH—such as occur with ischemia, anoxia, or cyanide poisoning—results in a rapid and massive loss of monoamines from storage vesicles into the neuronal cytoplasm. Contrary to usual depictions, vesicular stores of catecholamines and serotonin do not exist in a static state until exocytotic release. Rather, vesicular stores of monoamines exist in a highly dynamic equilibrium with the surrounding cytoplasm, with passive outward leakage of monoamines into the cytoplasm counterbalanced by inward active transport under the control of vesicular monoamine transporters (Figure 30-3). The magnitude and highly dynamic nature of this process can be appreciated by consideration of the effects of reserpine, a drug that blocks the ability of vesicular monoamine transporters to move monoamines from the cytoplasm into vesicles. Leakage of monoamines from vesicles is then no longer counterbalanced by vesicular translocation, and stores of monoamines are rapidly depleted. Monoamines share the acid environment of the storage granule matrix with adenosine triphosphate (ATP), peptides, and proteins, the best known of which are the chromogranins.247 The chromogranins are ubiquitous components of secretory vesicles; their widespread presence among endocrine tissues has led to their measurement in plasma as useful, albeit relatively nonspecific, markers of neuroendocrine tumors, including pheochromocytomas and carcinoid tumors.199 The process of exocytosis occurs at specialized locations on nerve endings or sympathetic varicosities dictated by the cell surface expression of specialized docking proteins that interact with other proteins on the surface of secretory vesicles.20 The process is stimulated by an influx of Ca2+, which in neurons is primarily controlled by nerve impulse–mediated membrane depolarization, and in adrenal medullary cells by acetylcholine release from innervating splanchnic nerves. The wide variety of voltage-, receptor-, G-protein–, and second messenger–operated Ca2+ channels provide numerous points for regulation of Ca2+-triggered exocytosis. Consequently, a variety of peptides, neurotransmitters, and humoral factors provide additional mechanisms for stimulation of exocytosis or may act to modulate nerve impulse–stimulated release of monoamines. Dopamine, norepinephrine, and serotonin also modulate their own release through occupation of autoreceptors. Regulation of monoamine release and synthesis is closely coordinated, thereby ensuring appropriate replenishment of the amines lost through exocytosis.193 Because the enzymes responsible for metabolism of catecholamines have intracellular locations, the primary mechanism limiting the life span of catecholamines in the extracellular space is uptake by active transport, not metabolism by enzymes (see Figure 30-3). Uptake is facilitated by transporters that belong to two large families of proteins with mainly neuronal or extraneuronal locations.62 Neuronal uptake of monoamines involves the dopamine transporter at dopaminergic neurons, the norepinephrine transporter at noradrenergic neurons, and the serotonin transporter at serotonergic neurons. These same transporters are also present at some non-neuronal locations, including adrenal chromaffin cells, endothelial cells of the lungs, specialized cells of the gastrointestinal tract, and some blood cells such as platelets. However, most extraneuronal uptake of monoamines is facilitated by a second set of proteins belonging to the organic cation transporter family. These latter transporters are expressed exclusively at extraneuronal locations and act on a broader range of substrates than the plasma membrane monoamine transporters expressed at neuronal locations. In addition to terminating the actions of released monoamines, the plasma membrane monoamine transporters at neuronal locations function in sequence with vesicular monoamine transporters to recycle catecholamines for re-release (see Figure 30-3). Thus most of the norepinephrine released and recaptured by sympathetic nerves is sequestered back into storage vesicles, thereby substantially reducing the requirements for synthesis of new transmitter. Metabolism of catecholamines occurs by a multiplicity of pathways catalyzed by an array of enzymes, resulting in a wide variety of metabolites (Figure 30-4).67 Deamination of catecholamines by monoamine oxidase (MAO) yields reactive aldehyde intermediates that are further metabolized to deaminated acids by aldehyde dehydrogenase, or to deaminated glycols by aldehyde or aldose reductase. The aldehyde intermediate formed from dopamine is a good substrate for aldehyde dehydrogenase, but not aldehyde or aldose reductase. In contrast, the aldehyde intermediate formed from the β-hydroxylated catecholamines—norepinephrine and epinephrine—is a good substrate for aldehyde or aldose reductase, but is a poor substrate for aldehyde dehydrogenase. Therefore, norepinephrine and epinephrine are preferentially deaminated to 3,4-dihydroxyphenylglycol (DHPG), the alcohol metabolite. Deamination to the deaminated acid metabolite, 3,4-dihydroxymandelic acid (DHMA), is not a favored pathway. Catechol-O-methyltransferase (COMT) is responsible for the second major pathway of catecholamine metabolism, catalyzing O-methylation of dopamine to methoxytyramine, norepinephrine to normetanephrine, and epinephrine to metanephrine. COMT is not present in monoamine-producing neurons, which contain exclusively MAO, but is present along with MAO in most extraneuronal tissues. The membrane-bound isoform of COMT, which has high affinity for catecholamines, is especially abundant in adrenal chromaffin cells. As a result of the preceding and other differences in the expression of metabolizing enzymes, catecholamines produced at neuronal and adrenal medullary locations follow different neuronal and extraneuronal pathways of metabolism (Figure 30-5). DHPG is further O-methylated by COMT in non-neuronal tissues to 3-methoxy-4-hydroxyphenylglycol (MHPG), a metabolite also produced to a limited extent by deamination of normetanephrine and metanephrine (see Figure 30-5). The latter O-methylated metabolites are produced in much smaller amounts compared with DHPG, and only at extraneuronal locations; their single largest source is adrenal chromaffin cells, which account for more than 90% of circulating metanephrine and 24 to 40% of circulating normetanephrine.56 Within the adrenals, normetanephrine and metanephrine are produced in a similar manner to the production of DHPG within sympathetic nerves, from norepinephrine and epinephrine leaking from storage granules into the chromaffin cell cytoplasm. The MHPG produced from DHPG and metanephrines may be sulfate-conjugated or metabolized to vanillylmandelic acid (VMA), the latter pathway catalyzed by the sequential actions of hepatic alcohol dehydrogenase and aldehyde dehydrogenase. At least 90% of the VMA formed in the body is produced in the liver, mainly from hepatic uptake and metabolism of circulating DHPG and MHPG.57 In contrast to production of VMA, production of homovanillic acid (HVA) from dopamine depends mainly on O-methylation of the deaminated metabolite of dopamine, 3,4-dihydroxyphenylacetic acid (DOPAC), and to a lesser extent on deamination of methoxytyramine, the O-methylated metabolite of dopamine (see Figure 30-4). As a result, HVA is formed in multiple tissues, with about 30% of circulating and urinary HVA arising from mesenteric organs and up to 20% from the brain. With the exception of VMA, all catecholamines and their metabolites are metabolized to sulfate conjugates by a specific sulfotransferase isoenzyme (SULT1A3). In humans, a single amino acid substitution confers the enzyme with particularly high affinity for dopamine and the O-methylated metabolites of catecholamines, including normetanephrine, metanephrine, and methoxytyramine.39 The SULT1A3 isoenzyme is found in high concentrations in gastrointestinal tissues, which therefore represent a major source of sulfate conjugates. In humans, VMA and the sulfates and glucuronide conjugates of MHPG represent the main end products of norepinephrine and epinephrine metabolism (Table 30-1). HVA and conjugates of HVA are the main metabolic end products of dopamine metabolism. These end products and the other conjugates are eliminated mainly by urinary excretion. As a result, their circulatory clearance is slow and plasma concentrations are high relative to those of the precursor amines. TABLE 30-1 Average Urinary Excretion of Catecholamines and Metabolites* *Average values in human subjects derived from published data.138 †Percent derived from norepinephrine and epinephrine. ‡Urinary free dopamine is derived mainly from renal decarboxylation of circulating L-dopa. Serotonin is not a substrate for COMT and follows simpler pathways of metabolism than those for catecholamines (Figure 30-6). Deamination of serotonin to the aldehyde intermediate is preferentially followed by oxidation to 5-hydroxyindoleacetic acid (5-HIAA) catalyzed by aldehyde dehydrogenase. Reduction to 5-hydroxytryptophol (5-HTOL) normally represents a relatively minor pathway, so that the major urinary excretion product of serotonin metabolism is 5-HIAA. Metabolism through these pathways is altered by consumption of ethyl alcohol, which through changes in redox state leads to increased formation of 5-HTOL. Increases in ratios of 5-HTOL to 5-HIAA in urine thereby provide a marker of alcohol abuse.41 The physiologic and behavioral actions of catecholamines and serotonin provide a foundation for understanding disorders of monoamine excess or deficiency. Major sites of catecholamine and serotonin synthesis and action are listed in Table 30-2 for both central and peripheral nervous systems. The table provides an anatomic framework showing the locations of adrenergic, dopaminergic, and serotonergic systems and varying modes of signal transmission by the monoamines. Catecholamines and serotonin regulate physiologic events at the cellular level through interaction with families of cell surface receptors. Adrenergic receptors, classified as α1 (subtypes α1a,b,d), α2 (subtypes α2a,b,c), β1, β2, and β3 subcategories, initiate cellular events in response to epinephrine and norepinephrine. Compared with norepinephrine, epinephrine demonstrates greater or equal affinity for α1 and α2 receptors, and equivalent potency for β1 receptors. For β2 receptor activation, epinephrine is 10- to 50-fold more potent than norepinephrine, and norepinephrine is 10-fold more active toward β3 receptors.113 Dopamine has known pharmacologic reactivity with α1– and α2-adrenergic receptors but transmits central and peripheral nerve signals primarily by selective interaction with a family of dopaminergic receptors designated as D1, D2, D3, D4, and D5 subtypes.178 An even larger family of serotonergic receptor subtypes (5-HT1A,B,D,E,F, 5-HT2A-C, 5-HT3, 5-HT4, 5-HT5A-B, 5-HT6, 5-HT7) have been identified by histologic and molecular techniques.118 Receptors, including α2-adrenergic 5-HT1A, 5-HT1B, and 5-HT1D, may serve as autoreceptors by turning off the release of their respective neurotransmitters via a negative feedback mechanism. The major physiologic effects of catecholamines and serotonin are explained in part by these diverse receptor interactions, which occur in function-specific locations throughout the vasculature and organ systems of the body. Most norepinephrine synthesis in the brain takes place in the locus coeruleus of the pons and in the reticular formation of the medulla oblongata. About half of the norepinephrine is produced in the locus coeruleus by only a small nucleus of neurons capable of responding in unison to sensory input signals.194 Norepinephrine-producing neurons in the lower brain stem send highly diffuse axonal projections throughout the brain as high as the cerebral cortex. They also send descending fibers to the spinal cord, where they synapse with preganglionic sympathetic neurons that communicate with the peripheral sympathetic nervous system. The norepinephrine-producing neurons of the brain stem process incoming sensory signals. They also participate in regulating the activity of the sympathetic nervous system.208 The locus coeruleus serves as part of the brain’s redundant arousal system. Neuronal discharge of norepinephrine from this brain stem region stimulates cortical activation, in addition to behavioral arousal, that occurs via increased muscle tone.124,125 The stimulant effect of amphetamine can be explained by the pharmacologic enhancement of norepinephrine release with associated cortical and muscle stimulation. A predominant physiologic function of norepinephrine systems in the brain is response to stress. Stress-induced stimuli activate brain stem production of norepinephrine with synaptic release and neuroactivation throughout many areas of the brain and spinal cord. Dopamine accounts for more than half the catecholamine production of the brain, but its distribution and functions are markedly different from those of norepinephrine. Dopaminergic neurotransmission is involved in processing sensory signals and in regulating hormonal release. Dopaminergic neurons in the retina and olfactory bulb have ultrashort projections that transmit signals within these neuronal centers for vision and smell. Neurons in the arcuate nucleus of the hypothalamus have projecting fibers that release dopamine in the portal vessels, exerting key neuroendocrine inhibition on prolactin and thyroid stimulating hormone release, as well as effects on other hormones of the pituitary gland.251 Dopamine is also produced by neurons in the substantia nigra and the ventral tegmentum. The ventral tegmental area modulates diverse forebrain functions such as cognitive activity through axonal projections to the prefrontal cortex and reward seeking behavior via axonal fibers sent to limbic structures of the brain.231 Consequently, disturbances in dopamine production and release from these areas of the brain are involved in several important neurologic and psychiatric disorders, with dopamine receptors serving as key pharmacologic targets for antipsychotic drugs.201 In addition, dopaminergic neurons in the substantia nigra project into the striatum to form the nigrostriatal system, which regulates the initiation and maintenance of motor function. Control by this system over extrapyrimidal (involuntary) movement is evidenced by loss of motor control when nigrostriatal neurons degenerate in Parkinson’s disease.200 Serotonin, similar to norepinephrine, is produced by small clusters of neurons in the brain stem regions, but serves a diverse range of behavioral and physiologic functions.245 Neurons in the raphe nuclei of the pons and upper brain stem are a primary source of brain serotonin and project axonal fibers throughout the brain and spinal cord. Physiologic and behavioral processes that may be influenced by this extensive serotoninergic system include memory, learning, feeding behavior, sleep patterns, thermoregulation, pain modulation, cardiovascular function, and hypothalamic regulation of pituitary hormones. Each of the 14 known subtypes of serotonin receptors have distinct patterns of distribution in the brain and account for diverse cognitive, behavioral, and physiologic responses.4,124 Raphe projections to the subcortical regions of the cerebral hemisphere (amygdala and hippocampus) activate receptors that influence mood and anxiety. Development of numerous pharmacologic agents for manipulation of serotonin concentrations and selective receptor responses in the brain clearly indicates the importance of the central serotoninergic system as a drug target.126 Increased synaptic concentrations of serotonin in the brain produce a quiet satiated waking state, providing a therapeutic target for anxiolytic and antidepressant drugs. Buspirone, for example, reduces anxiety through activation of serotonin autoreceptors in the subcortical areas of the brain, thus reducing serotonin release. Hypoactivity of the serotoninergic system, on the other hand, plays an important role in depression. Selective serotonin reuptake inhibitors such as fluoxetine, paroxetine, and sertraline are effective agents in the treatment of this form of central serotoninergic deficiency. Serotonin is also involved in hormonal regulation. Serotonin-releasing nerve fibers that originate in the raphe nuclei and terminate in the hypothalamus exert a circadian influence on pituitary-adrenal function by stimulating hypothalamic corticotropin-releasing hormone, as well as by signaling other hormonal events mediated by enhanced prolactin and growth hormone release.251 Serotonin also influences overall blood flow to the brain as evidenced by the vasoconstrictor effects of the amine on carotid vasculature and the beneficial pharmacologic effects of a serotoninergic agonist, sumatriptan, in the treatment of migraine. Norepinephrine is the principal neurotransmitter of the sympathetic branch of the autonomic nervous system. Sympathetic nerve transmission operates below the level of consciousness in controlling the physiologic functions of many organs and tissues of the body (Figure 30-7). The sympathetic nervous system plays a particularly important role in regulating cardiovascular function in response to postural, exertional, thermal, and mental stress. With sympathetic activation, heart rate is increased, peripheral arterioles are constricted, skeletal arterioles are dilated, and blood pressure is elevated. In addition, sympathetic nerve stimulation dilates pupils, inhibits smooth muscles of the intestines, bronchi, and bladder, and closes the sphincters. Sympathetic signals work in balance with the parasympathetic branch of the autonomic nervous system to maintain a stable internal environment. The hypothalamus, which integrates the autonomic and neuroendocrine systems, has a controlling influence over sympathetic outflow from brain stem centers and the spinal cord. Afferent or incoming sensory signals originate from pressure, stretch, chemical, pain, and temperature receptors located in visceral organs and vessels. Afferent nerve impulses enter the central nervous system to form the efferent component of local reflex arcs within the spinal cord or to ascend to higher centers such as the hypothalamus, where afferent signals are integrated with other neural pathways. Efferent or outgoing responses are transmitted by preganglionic sympathetic neurons that exit the spinal cord and converge on sympathetic ganglia chains along the spinal column or in visceral ganglia (see Figure 30-7). These preganglionic neurons release the neurotransmitter acetylcholine to regulate multiple postganglionic projections. Terminal branches of postganglionic fibers that project from these ganglia into target organs have varicosities that form a rich ground plexus for synaptic contact with a large number of effector cells in glands and muscle fibers. Most sympathetic postganglionic nerves liberate norepinephrine as their neurotransmitter. In limited locations, sympathetic nerve endings release acetylcholine, such as sweat glands that undergo a noradrenergic-to-cholinergic switch during development.229 Preganglionic sympathetic fibers also innervate the adrenal medulla, stimulating release of epinephrine from chromaffin cells. The relative contributions of individual organs to overall sympathetic activity have been inferred from data on the release of norepinephrine into plasma.3,56,73 Plasma norepinephrine is derived primarily from postganglionic sympathetic neurons with little contribution from the central nervous system. In the resting state, the overall rate of norepinephrine spillover into the systemic circulation is on the order of 200 to 600 ng/min. Because of intervening neuronal and extraneuronal removal processes, this spillover represents less than 10% of the total norepinephrine released by sympathetic nerves. Turnover of norepinephrine, representing loss due mainly to metabolism, is driven primarily in the resting state by leakage of norepinephrine from vesicles into the neuronal axoplasm.68 The physiologic response to sympathetic activity in individual organs is dependent not only on the rate of neurotransmitter release from postganglionic nerve varicosities but also on the types of adrenergic receptors and their locations in tissues. Vascular and organ-specific responses are classified in Table 30-3 according to interactions with adrenergic receptors. In peripheral target organs, α1– and β1-adrenergic receptors appear strategically located in the immediate vicinity of nerve terminals for rapid postsynaptic smooth muscle contraction, secretion, and endocrine activation via signals from the brain. Postsynaptic β2-adrenergic receptors in the heart also allow rapid sympathetic neuroactivation. Extrajunctional α2 and β2 receptors that are remote from sympathetic nerve terminals in vascular smooth muscle or platelets may be preferentially influenced by circulating catecholamines such as epinephrine produced by the adrenal gland.113 TABLE 30-3 Direct Effects of Adrenergic Activation on Some Organ Systems Based on Receptor Subtype E, Epinephrine; NE, norepinephrine. Modified from published data.143 The distribution of the six α1 (α1a,b,d) and α2 (α2a,b,c) subtypes is not completely understood and therefore is not included in the table. For receptor locations in the central nervous system, refer to von Bohlen und Halbach O, Dermietzel R. Neurotransmitters and neuromodulators: handbook of receptors and biological effects. Darmstadt: Wiley-VCH Verlag GmbH, 2002.256 An earlier concept of the sympathetic nervous system functioning primarily as an all-or-none responder to fight or flight has been challenged by studies indicating organ-specific responses to sympathetic activation.146,186 Thermal and pain sensors, for example, result in a sympathetic response that differs markedly from the physiologic response to baroreceptor signals produced by changes in blood pressure. An increase in environmental temperature reduces sympathetic tone in peripheral vascular beds, thereby resulting in vasodilatation and increased heat loses by radiation from the surface of the body. Pain receptor stimulation from surgical incision in anesthetized humans causes a proportionate increase in cutaneous vasoconstriction.234 During changes from supine to upright posture, however, baroreceptors signal both cardiac and vascular responses via the sympathetic nerves. In the heart, sympathetic release of norepinephrine increases the rate and force of cardiac muscle contractions, ultimately increasing cardiac output. In skin and mucosa, sympathetic activation causes vasoconstriction with a resultant increase in blood pressure and redistribution of blood to visceral organs. Therefore, although global sympathetic activation clearly operates in extreme conditions of stress, the differential control of sympathetic output enables an appropriate patterning of physiologic responses according to different behavioral and environmental stressors. Although often considered a part of the sympathetic nervous system, the adrenal medulla produces and secretes a different catecholamine, epinephrine, with different functions from the norepinephrine secreted by sympathetic nerves.64 The adrenal medulla and sympathetic nerves are also regulated separately, often in divergent directions in response to different forms of stress. This makes it appropriate to consider the two systems separately. Epinephrine released from the adrenals is more important as a metabolic than as a hemodynamic regulatory hormone.38 In particular, epinephrine stimulates lipolysis, ketogenesis, thermogenesis, and glycolysis and raises plasma glucose concentrations by stimulating glycogenolysis and gluconeogenesis. Epinephrine also has potent effects on pulmonary function, causing β2-adrenoceptor–mediated dilatation of airways. Circulating norepinephrine, in minor part derived from the adrenal medulla and functioning as a hormone, may have additional metabolic actions, but appears to have little importance for cardiovascular regulation compared to the higher concentrations of the amine at sympathoneuroeffector sites. Despite the apparent importance of the adrenal medulla in homeostasis, particularly in the regulation of metabolism, the medulla in contrast to the adrenal cortex is not vital for survival. Studies in adrenalectomized subjects clearly show that both hemodynamic and glucose counter-regulatory responses to insulin hypoglycemia, exercise, and other manipulations remain intact, despite the absence of epinephrine responses.112 This contrasts with the severe disturbances of blood pressure regulation that accompany loss of sympathetic nerves. In contrast to the sympathetic nervous system, the adrenal medulla makes a relatively minor contribution to the overall production and turnover of catecholamines (Table 30-4). However, because PNMT is expressed mainly in adrenal chromaffin cells, more than 90% of circulating epinephrine is derived from the adrenal medulla. This contrasts with circulating norepinephrine, more than 90% of which is derived from sympathetic nerves. TABLE 30-4 Contribution of the Adrenals to Circulating Catecholamines and Metabolites *Values represent rates of entry (spillovers) of catecholamines and metabolites into the venous drainage of the adrenal glands derived from clinical studies involving sampling of adrenal venous blood.56 †Values represent spillovers of catecholamines and metabolites into the circulation from all organs and tissues of the bodies derived from clinical studies involving regional blood sampling and kinetics analyses.56,57,96 Dopamine usually is thought of as a neurotransmitter in the brain or as an intermediate in the production of norepinephrine and epinephrine in the periphery. It has been presumed that these sources account for the large quantities of dopamine and dopamine metabolites excreted in urine. However, the contribution of the brain to circulating concentrations and urinary excretion of dopamine metabolites is now known to be relatively minor.67 Also, the dopamine formed in sympathetic nerves and in the adrenal medulla is mainly converted to norepinephrine. Most of the dopamine and dopamine metabolites in the circulation and excreted into urine are therefore derived from other sources (Figure 30-8). Accumulating evidence indicates the presence of a third peripheral catecholamine system, in which dopamine functions not as a neurotransmitter or circulating hormone, but as an autocrine or paracrine substance.92 In the kidneys, dopamine is now an established autocrine and/or paracrine effector substance contributing to the regulation of sodium excretion.24 Unlike neuronal catecholamine systems, the production of dopamine in the kidneys is largely independent of local synthesis of L-dopa by tyrosine hydroxylase. Thus, renal denervation does not affect urinary dopamine excretion. Instead, production of dopamine in the kidneys depends mainly on uptake by proximal tubular cells of L-dopa from the circulation (see Figure 30-8). The L-dopa is then converted to dopamine by aromatic amino acid decarboxylase, the activity of which is upregulated by a high-salt diet and downregulated by a low-salt diet. The presence of a renal dopamine paracrine/autocrine system explains the considerable amount of free dopamine excreted in the urine.19 Most derives from renal uptake and decarboxylation of circulating L-dopa and reflects the plasma concentrations of this amino acid and the function of the renal dopamine paracrine/autocrine system. Although the kidneys represent the major source of urinary free dopamine, this source does not account for the larger quantities of excreted dopamine metabolites such as HVA and dopamine sulfate. Findings of large arterial-to-portal venous increases in plasma concentrations of dopamine and its metabolites have indicated that substantial amounts of dopamine are produced and metabolized in the GI tract and other mesenteric organs.59 The substantial production and metabolism of dopamine in the human GI tract appear to reflect functions of dopamine as an enteric neuromodulator or paracrine/autocrine substance. Dopamine and dopamine receptor agonists stimulate bicarbonate secretion and protect against ulcer formation, whereas dopamine antagonists augment secretion of gastric acid and promote ulcer development.81 Dopamine also appears to influence (1) GI motility, (2) sodium transport, and (3) gastric and intestinal submucosal blood flow. In the pancreas, dopamine may modulate the secretion of digestive enzymes and bicarbonate. Morphologic studies have demonstrated the presence of cells in the GI tract that contain dopamine and express components of dopamine signaling pathways, including catecholamine biosynthetic enzymes and specific dopamine receptors and transporters.175 In the stomach, tyrosine hydroxylase is expressed in epithelial cells, including acid-secreting parietal cells. In the small intestine, cells of the lamina propria, including immune cells, also express tyrosine hydroxylase. The enzyme is additionally found in pancreatic exocrine cells. Consumption of food increases plasma concentrations of L-dopa, dopamine, and dopamine metabolites, particularly dopamine sulfate, indicating that dietary constituents may represent an important source of peripheral dopamine.50,94 Such a source does not, however, account for the substantial amounts of dopamine produced in peripheral tissues outside the digestive tract, or for that produced in digestive tissues of fasting individuals. In particular, plasma concentrations of both L-dopa and dopamine sulfate remain high, even after a 3-day fast. It is now clear that dopamine sulfate is produced mainly in the GI tract from both dietary and locally synthesized dopamine. This is consistent with findings that the GI tract contains high concentrations of the sulfotransferase isoenzyme, SULT1A3. Production of sulfate conjugates in the digestive tract appears to provide an enzymatic gut-blood barrier, for detoxifying dietary biogenic amines and delimiting physiologic effects of locally produced dopamine. The ENS is connected to the central nervous system by extrinsic parasympathetic and sympathetic motor neurons, and by extrinsic spinal and vagal sensory neurons. Through these bidirectional connections, the ENS can be monitored and modified.98,102 Despite the presence of these extrinsic nerve connections, the ENS can function autonomously in some intestinal regions. More than 95% of the body’s serotonin is located within the GI tract, and most is synthesized and stored in enterochromaffin cells in the gut mucosa. Serotonin is released from these cells in response to mechanical or chemical stimuli, such as the passage of food; this in turn stimulates both intrinsic (via 5-HT1P and 5-HT4 receptors) and extrinsic (via 5-HT3) vagal sensory nerve fibers.86 Intrinsic sensory neurons activated by serotonin stimulate the peristaltic reflex and secretion, whereas extrinsic sensory neurons initiate bowel sensations such as nausea, vomiting, abdominal pain, and bloating. The paracrine actions of serotonin are terminated by uptake into epithelial cells by the same serotonin transporter present in serotonergic neurons. Serotonin modulates numerous physiologic and behavioral systems in the human body and is involved in a wide variety of clinical disorders. In the ENS, for example, serotonin plays pivotal roles in the pathogenesis of the carcinoid syndrome and the irritable bowel syndrome.37,117 Differences in serotonin receptor subtypes provide a strong rationale for using pharmaceutical agents that selectively act on serotonin receptors in the treatment of these clinical disorders. Pheochromocytomas are catecholamine-producing neuroendocrine tumors arising from chromaffin cells of the adrenal medulla or extra-adrenal paraganglia.157 Those arising from extra-adrenal chromaffin tissue are referred to as extra-adrenal pheochromocytomas or paragangliomas. The same term, paragangliomas, is also used to refer to tumors arising from parasympathetic tissue in the head and neck, most of which do not produce significant quantities of catecholamines. About 85% of pheochromocytomas arise from the adrenal medulla, and about 15% arise from extra-adrenal chromaffin tissue (paragangliomas). Pheochromocytomas and catecholamine-producing paragangliomas are treacherous tumors that almost invariably cause devastating cardiovascular complications and death if not recognized and properly treated. Autopsy studies continue to indicate that most pheochromocytomas remain undetected throughout life, contributing to premature death.172 In many cases, presenting signs and symptoms may be misdiagnosed as another condition (e.g., preeclampsia in the pregnant patient with hypertension) with tragic consequences.167 To think of the tumor therefore remains the critical first step in diagnosis. The presence of a pheochromocytoma is usually suspected because of signs and symptoms that reflect the biological effects of catecholamines released by the tumor. Hypertension is the most common sign and can be sustained or paroxysmal. Symptoms include (1) headache, (2) palpitations, (3) diaphoresis, (4) pallor, (5) dyspnea, (6) nausea, (7) attacks of anxiety, and (8) generalized weakness. Although headache, palpitations, and sweating are nonspecific symptoms, their presence with hypertension should arouse immediate suspicion of the tumor. Signs and symptoms that occur in paroxysms reflect episodic catecholamine secretion. Paroxysmal attacks usually last less than an hour with intervals between attacks varying widely; these attacks may be as infrequent as once every few months. Pronounced but transient symptoms usually accompany such attacks; symptoms usually are less pronounced when hypertension is sustained. For additional details on the clinical presentation and other aspects of pheochromocytoma, the reader is referred to the texts of Manger and Gifford165 and Pacak and colleagues.204 Mutation testing, now routinely available for many of the above genes, has indicated that germline mutations are responsible for 30% or more of all pheochromocytomas—well in excess of the 10% of tumors previously thought to be hereditary. Between 12% and 24% of tumors with no obvious syndrome or family history appear to be due to otherwise unsuspected germline mutations.1,195 Mutation testing therefore should be considered in all patients with pheochromocytoma, independently of the presence of any obvious syndrome or family history. Conversely, patients with identified germline mutations should undergo routine periodic screening for pheochromocytoma, independently of the presence of signs and symptoms of catecholamine excess. Although most pheochromocytomas are benign, about 15% are malignant. A higher risk of malignant pheochromocytoma has been noted in patients with large or extra-adrenal primary tumors.66 The risk for malignancy is particularly significant in patients with mutations of the SDHB gene.1 Diagnosis of malignant pheochromocytoma is not possible based on histopathologic features, but instead requires evidence of metastatic lesions (e.g., in liver, lungs, lymphatic nodes, and bones). Metastases can occur more than 20 years after removal of an apparently benign solitary tumor. Therefore, all patients with a previous history of the tumor are at risk for recurrent or malignant disease and should undergo periodic screening for the tumor. Adrenal masses are present in 6% or more of the older population. Most are benign adenomas, but up to 10% represent pheochromocytomas. With escalation in the use of computed tomography and magnetic resonance imaging, an increasing frequency of incidental findings of adrenal masses has been reported during imaging procedures for unrelated conditions. These adrenal incidentalomas represent another situation in which biochemical testing for pheochromocytoma is recommended, irrespective of the presence of the usual signs and symptoms of the tumor.101 It is imperative that appropriate biochemical tests are employed for accurate diagnosis both once a tumor is suspected and in situations involving routine screening. Biochemical testing has traditionally relied on measurements of urinary catecholamines, metanephrines, and VMA.222 Most patients with hypertension and symptoms due to active pheochromocytomas have large increases in these analytes, making the tumor relatively easy to diagnose. Problems occur in those patients in whom hypertension is paroxysmal and in whom catecholamine secretion between episodes may be negligible. False-negative test results are also commonly encountered in patients with silent pheochromocytomas, in whom testing is carried out not because of signs or symptoms, but because of an adrenal incidentaloma or as part of a routine surveillance plan for recurrent or hereditary pheochromocytoma. In one study involving 35 patients with hereditary pheochromocytoma, rates of false-negative results for plasma and urinary catecholamines, urinary total metanephrines, and urinary VMA ranged from 29 to 53%; among the 35 patients, 6 (17%) had normal results on all four tests.61 Recognition that free metanephrines are produced by metabolism of catecholamines within chromaffin cells, including pheochromocytoma tumor cells, provided the rationale for development of these measurements as a new test to diagnose chromaffin cell tumors.63 Improved diagnostic performance of free metanephrines over other analytes is explained by several factors: (1) production of free metanephrines occurs secondary to leakage of catecholamines from storage vesicles into the chromaffin cell cytoplasm by a process that occurs continuously and independently of variations in catecholamine release by tumors; (2) normally only small quantities of metanephrines are produced in the body, and these compared to the parent amines are relatively unresponsive to sympathoadrenal activation; and (3) VMA and the metanephrines commonly measured in urine are different metabolites from the free metanephrines measured in plasma, and they are produced in different parts of the body by metabolic processes not directly related to the tumor itself. As reviewed elsewhere,99,203 the high diagnostic sensitivity of plasma free metanephrines has been confirmed by five independent groups. A sixth Australian group, reporting a diagnostic sensitivity for the test of 100%, has provided further independent support for superiority of the test over other available diagnostic tests.110 In the largest of all six independent studies—involving biochemical testing in more than 1000 patients, including 214 with pheochromocytoma—measurements of plasma free metanephrines and urinary fractionated metanephrines provided the highest diagnostic sensitivities156; however, measurements of plasma free metanephrines provided higher diagnostic specificity than measurements of urinary fractionated metanephrines. It is important to note that receiver operating characteristic curves show that a single test of plasma free metanephrines provided greater diagnostic efficacy than all other tests, even when carried out in combination (Figure 30-9). Based on these considerations, a now widely endorsed recommendation is that initial testing for pheochromocytoma should always include measurement of plasma free metanephrines or urinary fractionated metanephrines, or both.99,203 A lag has been reported in the shift from measurements of catecholamines to metanephrines,207,264 but this is expected, and measurements of metanephrines nevertheless are starting to supplant urinary and plasma catecholamines as first-line tests for the diagnosis of pheochromocytoma. Measurements of the parent catecholamines in urine or plasma remain important for providing supportive information, but a negative result for these tests cannot be used to exclude pheochromocytoma. In contrast, negative results for measurements of plasma free metanephrines reliably exclude almost all catecholamine-producing pheochromocytomas. Exceptions include small or microscopic (<1 cm) tumors in asymptomatic patients encountered during routine screening and tumors that synthesize predominantly dopamine. However, the latter tumors are extremely rare, have an unusual clinical presentation, and may be diagnosed by additional measurements of methoxytyramine, the O-methylated metabolite of dopamine.69 Increases in plasma free or urinary fractionated metanephrines are usually large enough to conclusively establish the presence of most cases of pheochromocytoma, but a major remaining problem involves test results in the “gray area,” which are not sufficiently elevated to reliably confirm pheochromocytoma (Figure 30-10). The problems involved in distinguishing false-positive from true-positive results are common to all biochemical tests of catecholamine excess; false-positive results must always be expected when the test reference interval is set at anything less than the 100% confidence intervals of a reference population. For pheochromocytoma, the large number of patients tested, very few of whom have the tumor, compounds this problem. The low pretest prevalence of pheochromocytoma means that false-positive biochemical results outnumber true-positive results, making it difficult to unequivocally confirm the tumor in the vast majority of patients with positive results. In such patients, additional biochemical testing is usually necessary. The shaded areas beyond the upper reference intervals shown in Figure 30-10 indicate the plasma concentrations or urinary outputs of metanephrines where positive results are of insufficient magnitude to allow false-positive and true-positive results to be reliably distinguished. Among patients with test results in the shaded area, follow-up testing is usually required to further confirm or exclude pheochromocytoma. Beyond the boundary of shaded areas, the probability of a catecholamine-producing tumor approaches 100%. The smaller shaded area in the figure for measurements of plasma free metanephrines compared with urinary fractionated metanephrines indicates that pheochromocytoma can be unequivocally confirmed in a larger proportion of patients using measurements of plasma metanephrines than using urinary metanephrines. This conversely implies that more follow-up testing is required after measurements of urinary fractionated metanephrines than after measurements of plasma free metanephrines. Before additional biochemical testing is initiated, consideration should be given to eliminating possible causes of false-positive results.65 Such results may occur because of inappropriate sampling conditions, such as blood sampled without an overnight fast or a preceding 20-minute period of supine rest.158 Medications leading to direct analytical interference or that influence the physiologic disposition of catecholamines and their metabolites represent other leading causes of false-positive results. Tricyclic antidepressants and phenoxybenzamine (dibenzyline) are particularly problematic, in one study accounting for 41% of all false-positive elevations in plasma normetanephrine, and for 44 to 45% of all false-positive elevations in urinary and plasma norepinephrine.65 Patterns of increases in plasma free metanephrines and catecholamines also are useful for confirming pheochromocytoma in patients for whom initial tests of free metanephrines are positive but insufficiently elevated for a firm diagnosis.65 More specifically, patients with pheochromocytoma usually have larger relative increases in metanephrines than in the parent catecholamines, whereas patients with false-positive results due to sympathoadrenal activation usually have larger increases in catecholamines than in metanephrines. When biochemical testing continues to yield equivocal results, the clonidine suppression test may be useful for further confirming or excluding pheochromocytoma. As originally introduced by Bravo and associates,17 this test was designed to distinguish patients with increases in plasma catecholamines due to pheochromocytoma from those with increases due to sympathetic activation. By activating α2-adrenoceptors in the brain and on sympathetic nerve endings, clonidine suppresses norepinephrine release by sympathetic nerves. Decreases in elevated plasma norepinephrine after clonidine therefore suggest sympathetic activation, whereas lack of a decrease suggests pheochromocytoma. A problem with the clonidine suppression test is that patients with normal or mildly increased plasma concentrations of norepinephrine may have clonidine-induced decreases in plasma norepinephrine, despite the presence of a pheochromocytoma.65,239 Such patients represent those in whom it is most difficult to conclusively diagnose pheochromocytoma. Additional measurements of plasma normetanephrine before and after clonidine overcome this limitation.65 In a study involving 48 patients with and 49 patients without pheochromocytoma, lack of decrease in and elevated plasma concentrations of norepinephrine or normetanephrine after clonidine confirmed pheochromocytoma with high specificity (98 to 100%). However, of 48 patients with pheochromocytoma, 16 had normal concentrations or decreases in norepinephrine after clonidine. In contrast, plasma normetanephrine remained elevated after clonidine in all but two patients, indicating higher sensitivity (96% vs. 67%) and more reliable diagnosis using normetanephrine than norepinephrine responses to clonidine. Box 30-1 outlines the clonidine suppression test protocol, along with these added testing recommendations.
Catecholamines and Serotonin
Chemical Structure
Biosynthesis, Release, and Metabolism
Biosynthesis
Storage and Release
Uptake and Metabolism
Physiology of Catecholamine and Serotonin Systems
Central Nervous System
Sympathetic Nervous System
Receptor Subtype (Relative Potency)
Tissue Location
Response
α1 (NE ≤ E)
Heart
Increased force
Arterioles
Constriction
Veins
Constriction
Pupils
Dilation
Adrenergic sweat glands
Secretion
GI sphincters
Contraction
Urinary bladder sphincter
Contraction
Uterus in pregnancy
Contraction
Spleen capsule
Contraction
Penis
Ejaculation
α2 (NE ≤ E)
Arterioles
Constriction
Adipocytes
Inhibit lipolysis
GI motility and tone
Decreased
Intestinal secretions
Inhibit
Pancreatic islet cell secretion
Inhibit
Platelet aggregation
Stimulate
β1 (NE = E)
Heart
Increased rate and force
Kidney
Renin release
Adipocytes
Stimulate lipolysis
β2 (NE E)
Heart
Increased rate and force
Arterioles
Dilation
Gallbladder and ducts
Relaxation
GI motility and tone
Decreased
Adipocytes
Stimulate lipolysis
Bronchial muscle
Dilation
β3 (NE > E)
Adipocytes
Stimulate lipolysis
Adrenal Medullary System
Peripheral Dopaminergic System
Enteric Nervous System
Clinical Applications
Pheochromocytoma
Catecholamines and Serotonin
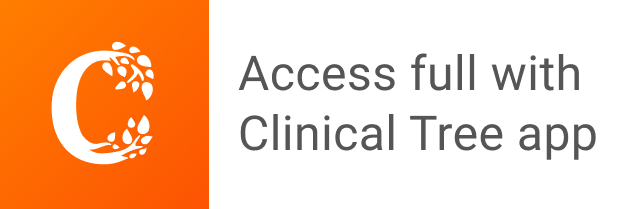