All cells use adenosine triphosphate (ATP) continuously and require a constant supply of fuels to provide energy for ATP generation. Insulin and glucagon are the two major hormones that regulate fuel mobilization and storage. Their function is to ensure that cells have a constant source of glucose, fatty acids, and amino acids for ATP generation and for cellular maintenance (Fig. 19.1).
FIGURE 19.1 Maintenance of fuel supplies to tissues. Glucagon release activates the pathways shown. ATP, adenosine triphosphate.
Because most tissues are partially or totally dependent on glucose for the generation of ATP and for the production of precursors of other pathways, insulin and glucagon maintain blood glucose levels near 70 to 100 mg/dL (90 mg/dL is the same as 5 mM), despite the fact that carbohydrate intake varies considerably over the course of a day. The maintenance of constant blood glucose levels (glucose homeostasis) requires these two hormones to regulate carbohydrate, lipid, and amino acid metabolism in accordance with the needs and capacities of individual tissues. Basically, the dietary intake of all fuels in excess of immediate need is stored, and the appropriate fuel is mobilized when demand occurs. For example, when dietary glucose is not available to cells in sufficient quantities, fatty acids are mobilized and used by skeletal muscle as a fuel (see Chapters 2 and 30). Under these circumstances, the liver can also convert fatty acids to ketone bodies that can be used by the brain. The fatty acids that are mobilized under these conditions spare glucose for use by the brain and other glucose-dependent tissues (such as red blood cells).
Insulin and glucagon are important for the regulation of fuel storage and fuel mobilization (Fig. 19.2). Insulin, released from the β-cells of the pancreas in response to carbohydrate ingestion, promotes glucose utilization as a fuel and glucose storage as fat and glycogen. Insulin, therefore, is a major anabolic hormone. In addition to its storage function, insulin increases protein synthesis and cell growth. Blood insulin levels decrease as glucose is taken up by tissues and utilized. Glucagon, the major insulin counterregulatory hormone, is decreased in response to a carbohydrate meal and elevated during fasting. Its concentration in the blood increases as circulating levels of glucose fall, a response that promotes glucose production via glycogenolysis (glycogen degradation) and gluconeogenesis (glucose synthesis from amino acids and other noncarbohydrate precursors). Increased levels of circulating glucagon relative to insulin also stimulate the mobilization of fatty acids from adipose tissue. Epinephrine (the fight-or-flight hormone) and cortisol (a glucocorticoid released from the adrenal cortex in response to fasting and chronic stress) have effects on fuel metabolism that oppose those of insulin. Therefore, epinephrine and cortisol are considered to be insulin counterregulatory hormones.
FIGURE 19.2 Insulin and its counterregulatory hormones. A. Insulin promotes glucose storage as TG or glycogen. B. Glucagon and epinephrine promote glucose release from the liver, activating glycogenolysis and gluconeogenesis. Cortisol will stimulate both glycogen synthesis and gluconeogenesis. TG, triglyceride.
Insulin and glucagon are polypeptide hormones synthesized as prohormones in the pancreatic β- and α-cells, respectively. Proinsulin is cleaved into mature insulin and a connection peptide (C-peptide) in storage vesicles and precipitated with Zn2+. Insulin secretion is regulated principally by changes in blood glucose levels. Glucagon is also synthesized as a prohormone and cleaved into mature glucagon within storage vesicles. Its release is regulated principally through changes in the level of glucose and insulin bathing the α-cells located in the pancreatic islets of Langerhans.
Glucagon exerts its effects on cells by binding to a receptor located on the plasma membrane of target cells for this hormone. The binding to these specific receptors by glucagon stimulates the synthesis of the intracellular second messenger, cyclic adenosine monophosphate (cAMP) (Fig. 19.3). cAMP activates protein kinase A (PKA), which phosphorylates key regulatory enzymes, thereby activating some while inhibiting others. Insulin, on the other hand, promotes the dephosphorylation of these key enzymes, leading to their activation or deactivation, depending on the enzyme. Changes in cAMP levels also induce or repress the synthesis of a number of enzymes.
FIGURE 19.3 Cellular response to glucagon, which is released from the pancreas in response to a decrease in blood glucose levels. cAMP, cyclic adenosine monophosphate.
Insulin binds to a receptor on the cell surface of insulin-sensitive tissues and initiates a cascade of intracellular events that differs from those stimulated by glucagon. Insulin binding activates both autophosphorylation of the receptor and the phosphorylation of other enzymes by the receptor’s tyrosine kinase domain (see Chapter 10, Section XII.B.1). The complete routes for signal transduction between this point and the final effects of insulin on the regulatory enzymes of fuel metabolism have not yet been fully established.
THE WAITING ROOM 
Deborah S. returned to her physician for her monthly office visit. She has been seeing her physician for over a year because of obesity and elevated blood glucose levels. She still weighed 198 lb, despite trying to adhere to her diet. Her blood glucose level at the time of the visit, two hours after lunch, was 221 mg/dL (reference range, 70 to 140 mg/dL). She suffers from type 2 diabetes, an impaired response to insulin. Understanding the actions of insulin and glucagon is critical for understanding this disorder.
Connie C. is a 46-year-old woman who 6 months earlier began noting episodes of fatigue and confusion in the morning before eating and sometimes after jogging. These episodes were occasionally accompanied by blurred vision and an unusually urgent sense of hunger. The ingestion of food relieved all of her symptoms within 25 to 30 minutes. In the last month, these attacks have occurred more frequently throughout the day, and she has learned to diminish their occurrence by eating between meals. As a result, she has recently gained 8 lb.
A random (nonfasting) serum glucose level done at 4:30 P.M. during her first office visit was subnormal at 61 mg/dL. Her physician, suspecting she was having episodes of hypoglycemia, ordered a series of fasting serum glucose, insulin, and C-peptide levels. In addition, he asked Connie C. to keep a careful diary of all the symptoms that she experienced when her attacks were most severe.
I. Metabolic Homeostasis
Living cells require a constant source of fuels from which to derive ATP for the maintenance of normal cell function and growth. Therefore, a balance must be achieved among carbohydrate, fat, and protein intake, their rates of oxidation, and their rates of storage when they are present in excess of immediate need. Alternatively, when the demand for these substrates increases, the rate of mobilization from storage sites and the rate of their de novo synthesis also require balanced regulation. The control of the balance between substrate need and substrate availability is referred to as metabolic homeostasis (Fig. 19.4). The intertissue integration required for metabolic homeostasis is achieved in three principal ways:
FIGURE 19.4 Metabolic homeostasis. The balance between fuel availability and the needs of tissues for different fuels is achieved by three types of messages: the level of the fuel or nutrients in the blood, the level of one of the hormones of metabolic homeostasis, or nerve impulses that affect tissue metabolism or the release of hormones.
Fatty acids provide an example of the influence that the level of a compound in the blood has on its own rate of metabolism. The concentration of fatty acids in the blood is the major factor determining whether skeletal muscles will use fatty acids or glucose as a fuel (see Chapter 30). In contrast, hormones are (by definition) intravascular carriers of messages between their sites of synthesis and their target tissues. Epinephrine, for example, is a flight-or-fight hormone that in times of stress signals an immediate need for increased fuel availability. Its level is regulated principally through the activation of the sympathetic nervous system.
Insulin and glucagon, however, are the two major hormones that regulate fuel storage and mobilization (see Fig. 19.2). Insulin is the major anabolic hormone of the body. It promotes the storage of fuels and the utilization of fuels for growth. Glucagon is the major hormone of fuel mobilization (Fig. 19.5). Other hormones, such as epinephrine, are released as a response of the central nervous system to hypoglycemia, exercise, or other types of physiologic stress. Epinephrine and other stress hormones also increase the availability of fuels (see Fig. 19.5). The major hormones of fuel homeostasis, insulin and glucagon, fluctuate continuously in response to our daily eating pattern.
FIGURE 19.5 Signals that regulate metabolic homeostasis. The major stress hormones are epinephrine and cortisol. ATP, adenosine triphosphate.
Glucose has a special role in metabolic homeostasis. Many tissues (e.g., the brain, red blood cells, kidney medulla, exercising skeletal muscle) are dependent on glycolysis for all or a part of their energy needs. As a consequence, these tissues require uninterrupted access to glucose to meet their rapid rate of ATP use. In the adult, a minimum of 190 g glucose is required per day: approximately 150 g for the brain and 40 g for other tissues. Significant decreases of blood glucose below 60 mg/dL limit glucose metabolism in the brain and may elicit hypoglycemic symptoms (as experienced by Connie C.), presumably because the overall process of glucose flux through the blood–brain barrier, into the interstitial fluid, and subsequently into the neuronal cells is slow at low blood glucose levels because of the Km values of the glucose transporters required for this to occur (see Chapter 21).
II. Major Hormones of Metabolic Homeostasis
The hormones that contribute to metabolic homeostasis respond to changes in the circulating levels of fuels that, in part, are determined by the timing and composition of our diet. Insulin and glucagon are considered the major hormones of metabolic homeostasis because they continuously fluctuate in response to our daily eating pattern. They provide good examples of the basic concept of hormonal regulation. Certain features of the release and action of other insulin counterregulatory hormones, such as epinephrine, norepinephrine, and cortisol, will be described and compared with insulin and glucagon.
Insulin is the major anabolic hormone that promotes the storage of nutrients: glucose storage as glycogen in liver and muscle, conversion of glucose to triacylglycerols in liver and their storage in adipose tissue, and amino acid uptake and protein synthesis in skeletal muscle (Fig. 19.6). It also increases the synthesis of albumin and other proteins by the liver. Insulin promotes the use of glucose as a fuel by facilitating its transport into muscle and adipose tissue. At the same time, insulin acts to inhibit fuel mobilization.
FIGURE 19.6 Major sites of insulin action on fuel metabolism. VLDL, very-low-density lipoprotein; ⊕, stimulated by insulin; ⊖, inhibited by insulin.
Glucagon acts to maintain fuel availability in the absence of dietary glucose by stimulating the release of glucose from liver glycogen (see Chapter 26), by stimulating gluconeogenesis from lactate, glycerol, and amino acids (see Chapter 28), and, in conjunction with decreased insulin, by mobilizing fatty acids from adipose triacylglycerols to provide an alternate source of fuel (see Chapter 30 and Fig. 19.7). Its sites of action are principally the liver and adipose tissue; it has no influence on skeletal muscle metabolism because muscle cells lack glucagon receptors. The message carried by glucagon is that “glucose is gone”; that is, the current supply of glucose is inadequate to meet the immediate fuel requirements of the body.
FIGURE 19.7 Major sites of glucagon action in fuel metabolism. ⊕, pathways stimulated by glucagon; ⊖, pathways inhibited by glucagon.
The release of insulin from the β–cells of the pancreas is dictated primarily by the level of glucose in the blood bathing the β–cells in the islets of Langerhans. The highest levels of insulin occur approximately 30 to 45 minutes after a high-carbohydrate meal (Fig. 19.8). They return to basal levels as the blood glucose concentration falls, approximately 120 minutes after the meal. The release of glucagon from the α–cells of the pancreas, conversely, is controlled principally through a reduction of glucose and/or a rise in the concentration of insulin in the blood bathing the α–cells in the pancreas. Therefore, the lowest levels of glucagon occur after a high-carbohydrate meal. Because all of the effects of glucagon are opposed by insulin, the simultaneous stimulation of insulin release and suppression of glucagon secretion by a high-carbohydrate meal provides integrated control of carbohydrate, fat, and protein metabolism.
FIGURE 19.8 Blood glucose, insulin, and glucagon levels after a high-carbohydrate meal.
Insulin and glucagon are not the only regulators of fuel metabolism. The intertissue balance between the use and storage of glucose, fat, and protein is also accomplished by the circulating levels of metabolites in the blood, by neuronal signals, and by the other hormones of metabolic homeostasis (epinephrine, norepinephrine, cortisol, and others) (Table 19.1). These hormones oppose the actions of insulin by mobilizing fuels. Like glucagon, they are insulin counterregulatory hormones (Fig. 19.9). Of all these hormones, only insulin and glucagon are synthesized and released in direct response to changing levels of fuels in the blood. The release of cortisol, epinephrine, and norepinephrine is mediated by neuronal signals. Rising levels of the insulin counterregulatory hormones in the blood reflect, for the most part, a current increase in the demand for fuel.
TABLE 19.1 Physiologic Actions of Insulin and Insulin Counterregulatory Hormones
HORMONE | FUNCTION | MAJOR METABOLIC PATHWAYS AFFECTED |
Insulin |
|
|
Glucagon |
|
|
Epinephrine |
|
|
Cortisol |
|
|
FIGURE 19.9 Major insulin counterregulatory hormones. The stress of a low blood glucose level mediates the release of the major insulin counterregulatory hormones through neuronal signals. Hypoglycemia is one of the stress signals that stimulate the release of cortisol, epinephrine, and norepinephrine. ACTH is released from the pituitary and stimulates the release of cortisol (a glucocorticoid) from the adrenal cortex. Neuronal signals stimulate the release of epinephrine from the adrenal medulla and norepinephrine from nerve endings. Neuronal signals also play a minor role in the release of glucagon. Although norepinephrine has counterregulatory actions, it is not a major counterregulatory hormone. ACTH, adrenocorticotropic hormone.
III. Synthesis and Release of Insulin and Glucagon
A. Endocrine Pancreas
Insulin and glucagon are synthesized in different cell types of the endocrine pancreas, which consists of microscopic clusters of small glands, the islets of Langerhans, scattered among the cells of the exocrine pancreas. The α-cells secrete glucagon and the β-cells secrete insulin into the hepatic portal vein via the pancreatic veins.
B. Synthesis and Secretion of Insulin
Insulin is a polypeptide hormone. The active form of insulin is composed of two polypeptide chains (the A-chain and the B-chain) linked by two interchain disulfide bonds. The A-chain has an additional intrachain disulfide bond (Fig. 19.10).
FIGURE 19.10 Cleavage of proinsulin to insulin. Proinsulin is converted to insulin by proteolytic cleavage, which removes the C-peptide and a few additional amino acid residues. Cleavage occurs at the arrows. (Republished with permission of McGraw Hill LLC from Murray RK, Granner DK, Mayes PA, et al. Harper’s Biochemistry. 23rd ed. Appleton & Lange; 1993:560; permission conveyed through Copyright Clearance Center, Inc.)
Insulin, like many other polypeptide hormones, is synthesized as a preprohormone that is converted in the rough endoplasmic reticulum (RER) to proinsulin. The “pre” sequence, a short hydrophobic signal sequence at the N-terminal end, is cleaved as it enters the lumen of the RER. Proinsulin folds into the proper conformation, and disulfide bonds are formed between the cysteine residues. It is then transported in microvesicles to the Golgi complex. It leaves the Golgi complex in storage vesicles, where a protease removes the biologically inactive “connecting peptide” (C-peptide) and a few small remnants, resulting in the formation of biologically active insulin (see Fig. 19.10). Zinc ions are also transported in these storage vesicles. Cleavage of the C-peptide decreases the solubility of the resulting insulin, which then coprecipitates with zinc. Exocytosis of the insulin storage vesicles from the cytosol of the β-cell into the blood is stimulated by rising levels of glucose in the blood bathing the β-cells.
Glucose enters the β-cell via specific glucose transporter proteins known as GLUT 2 (see Chapter 21). Glucose is phosphorylated through the action of glucokinase to form glucose 6-phosphate, which is metabolized through glycolysis, the tricarboxylic acid (TCA) cycle, and oxidative phosphorylation. These reactions result in an increase in ATP levels within the β-cell (circle 1 in Fig. 19.11). As the β-cell ATP/adenosine diphosphate (ADP) ratio increases, the activity of a membrane-bound, ATP-dependent K+ channel (K+ATP) is inhibited (i.e., the channel is closed) (circle 2 in Fig. 19.11). The closing of this channel leads to membrane depolarization (as the membrane is normally hyperpolarized, see circle 3 in Fig. 19.11), which activates a voltage-gated Ca2+ channel that allows Ca2+ to enter the β-cell such that intracellular Ca2+ levels increase significantly (Fig. 19.11, circle 4). The increase in intracellular Ca2+ stimulates the fusion of insulin containing exocytotic vesicles with the plasma membrane, resulting in insulin secretion (Fig. 19.11, circle 5). Thus, an increase in glucose levels within the β-cells initiates insulin release.
FIGURE 19.11 Release of insulin by the β-cells. Details are given in the text. ATP, adenosine triphosphate; TCA, tricarboxylic acid; ⊕, stimulation; ⊖, inhibition; Δψ, membrane potential.
Other intracellular metabolites, particularly nicotinamide adenine dinucleotide phosphate (NADPH), have been proposed to play important roles in insulin release in response to glucose. This will be discussed further in later chapters.
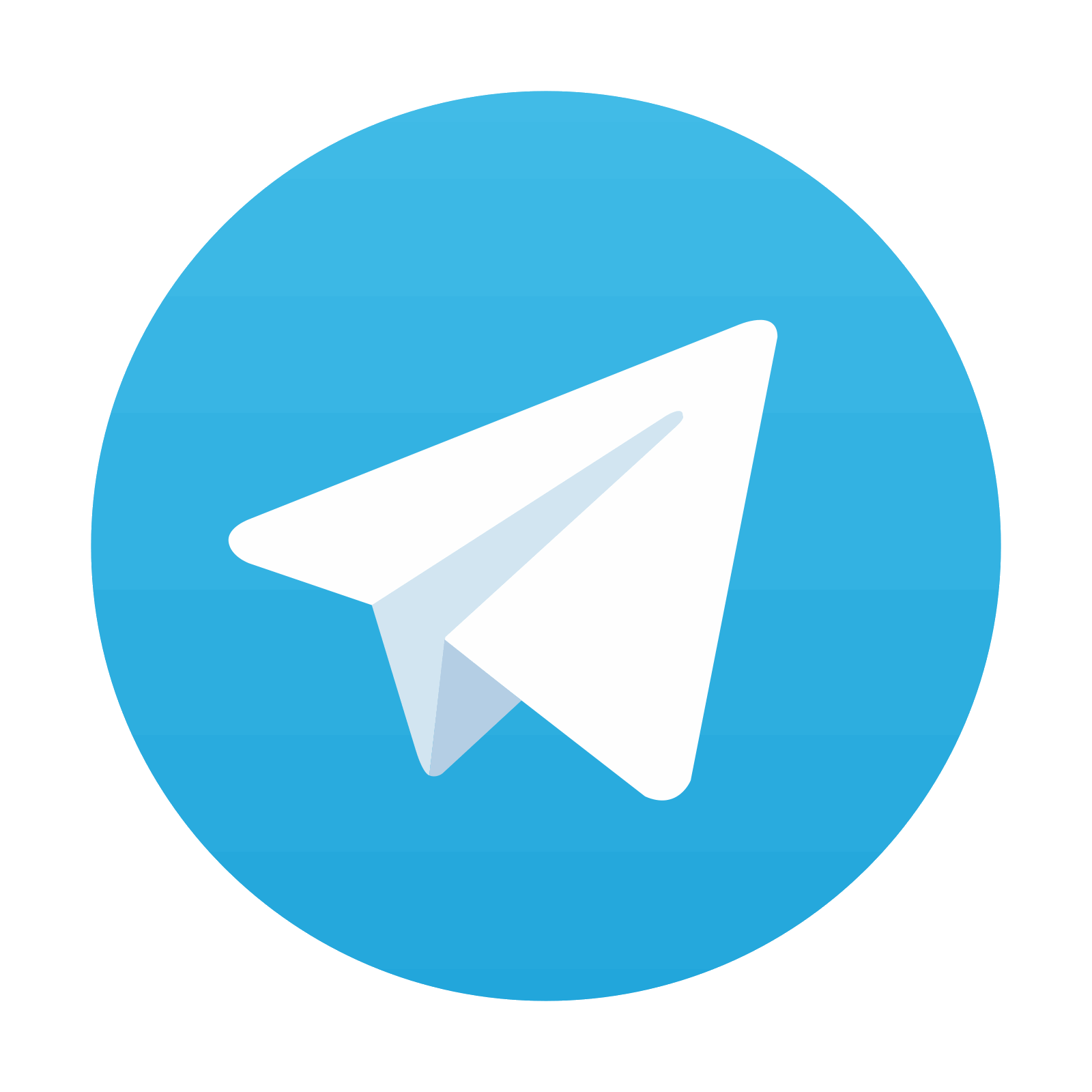
Stay updated, free articles. Join our Telegram channel
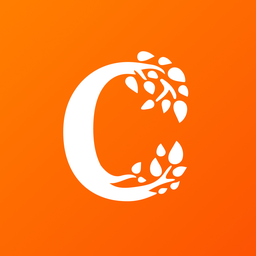
Full access? Get Clinical Tree
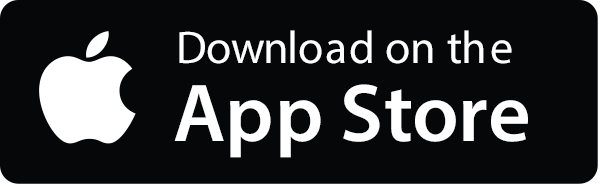
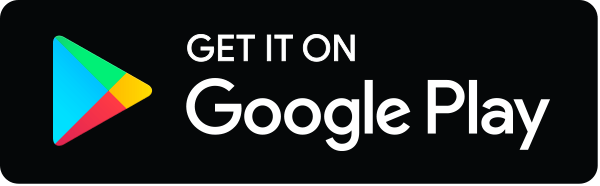