Chapter 8
Adaptive Immunity
Neal S. Rote and Kathryn L. McCance
The third line of defense in the human body is adaptive (acquired) immunity, often called the immune response or immunity.1 Once external barriers have been compromised and inflammation (see Chapter 7) has been activated, the adaptive immune response is called into action. The molecules and cells of the immune response are closely integrated with those of the innate response. Both systems are essential for complete protection against infectious disease: inflammation is relatively rapid, nonspecific, and short-lived, whereas adaptive immunity is slower acting, specific, and very long-lived. Thus, inflammation is the “first responder” that contains the initial injury and slows spread of infection, whereas adaptive immunity slowly augments the initial defenses against infection and provides long-term security against reinfection. The collaborative and beneficial nature of inflammation and adaptive immunity can, on occasion, fail. Chapter 9 discusses these medically relevant aberrations in both inflammation and immunity, including allergies, diseases that involve unwanted immunologic destruction of healthy tissue, and diseases that are caused by a deficiency in the normal immune or inflammatory responses. Chapter 10 presents an overview of infection and Chapter 11 discusses the connection between stress and disease and the interrelatedness of the immune, nervous, and endocrine systems.
General Characteristics of Adaptive Immunity
The immune system of the normal adult is continually challenged by a spectrum of substances that it may recognize as foreign, or “non-self.” These substances, called foreign antigens, are often associated with pathogens such as viruses, bacteria, fungi, or parasites, although they are also found on noninfectious environmental agents such as pollens, foods, and bee venom, and still others are associated with clinically derived drugs, vaccines, transfusions, and transplanted tissues (Table 8-1). Unlike inflammation, which is nonspecifically activated by damage to cells as well as by the action of pathogenic microorganisms, the immune response is primarily designed to afford long-term specific protection (i.e., immunity) against particular invading microorganisms; that is, it has a “memory” function. The products of the adaptive immune response include a type of serum protein—immunoglobulins, or antibodies—and a type of blood cell—lymphocytes (Figure 8-1).
TABLE 8-1
CLINICAL USE OF ANTIGEN OR ANTIBODY
ANTIGEN SOURCE | PROTECTION: COMBAT ACTIVE DISEASE | PROTECTION: VACCINATION | DIAGNOSIS | THERAPY |
Infectious agents | Neutralize or destroy pathogenic microorganisms (e.g., antibody response against viral infections) | Induce safe and protective immune response (e.g., recommended childhood vaccines) | Measure circulating antigen from infectious agent or antibody (e.g., diagnosis of hepatitis B infection) | Passive treatment with antibody to treat or prevent infection (e.g., administration of antibody against hepatitis A) |
Cancers | Prevent tumor growth or spread (e.g., immune surveillance to prevent early cancers) | Prevent cancer growth or spread (e.g., vaccination with cancer antigens) | Measure circulating antigen (e.g., circulating PSA for diagnosis of prostate cancer) | Immunotherapy (e.g., treatment of cancer with antibodies against cancer antigens) |
Environmental substances | Prevent entrance into body (e.g., secretory IgA limits systemic exposure to potential allergens) | No clear example | Measure circulating antigen or antibody (e.g., diagnosis of allergy by measuring circulating IgE) | Immunotherapy (e.g., administration of antigen for desensitization of individuals with severe allergies) |
Self-antigens | Immune system tolerance to self-antigens, which may be altered by an infectious agent leading to autoimmune disease (see Chapter 9) | Some cases of vaccination alter tolerance to self-antigens leading to autoimmune disease | Measure circulating antibody against self-antigen for diagnosis of autoimmune disease (see Chapter 9) | No clear example |
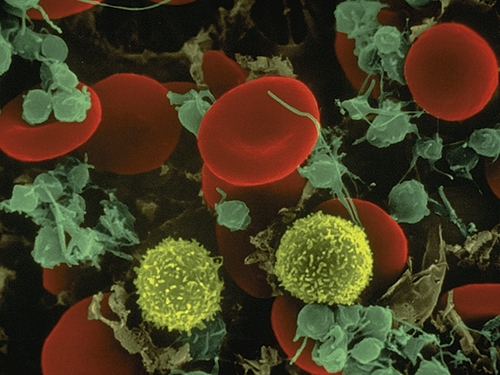
Specificity and memory are the primary characteristics that differentiate the immune response from other protective mechanisms. This chapter first discusses the nature of that specificity by defining the various types of antigens that may be seen by the immune system, the ways in which they are recognized by antibodies and lymphocytes, and the specific intercellular recognition molecules that are necessary for effective immune responses. After the recognition molecules are defined, the development of the immune response is discussed. An immune response can be divided into two phases (Figure 8-2). Before birth, humans produce a large population of T lymphocytes (T cells) and B lymphocytes (B cells) that have the capacity to recognize almost any foreign antigen found in the environment. Each individual T or B cell, however, specifically recognizes only one particular antigen, but the sum of the population of lymphocyte specificities may represent millions of foreign antigens. This process is called the generation of clonal diversity and occurs in specialized (primary) lymphoid organs—the thymus for T cells and the bone marrow for B cells. While passing through these tissues, the lymphocytes mature and undergo changes that commit them to becoming either B or T cells. Lymphocytes are released from these organs into the circulation as immature cells that have the capacity to react with antigen (immunocompetent). These cells migrate to other (secondary) lymphoid organs in the body in preparation for exposure to antigen (Figure 8-3).
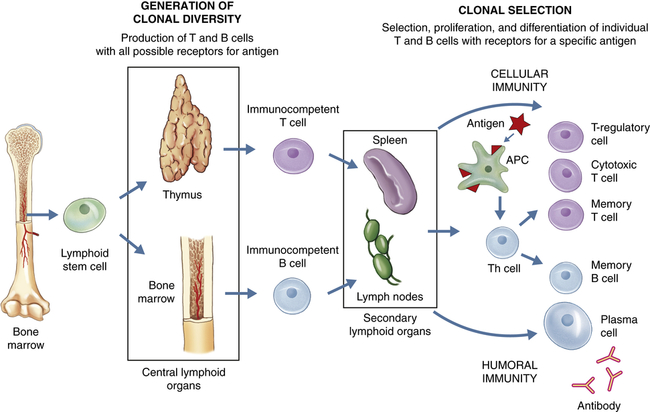
The immune response can be separated into two phases: the generation of clonal diversity and clonal selection. During the generation of clonal diversity, lymphoid stem cells from the bone marrow migrate to the central lymphoid organs (the thymus or regions of the bone marrow), where they undergo a series of cellular division and differentiation stages resulting in either immunocompetent T cells from the thymus or immunocompetent B cells from the bone marrow. (This process is outlined in more detail in Figures 8-10 and 8-12.) These cells are still naïve in that they have never encountered foreign antigen. The immunocompetent cells enter the circulation and migrate to the secondary lymphoid organs (e.g., spleen and lymph nodes), where they take up residence in B- and T-cell–rich areas. The clonal selection phase is initiated by exposure to foreign antigen. The antigen is usually processed by antigen-presenting cells (APCs) for presentation to helper T cells (Th cells) (more detail in Figure 8-16). The intercellular cooperation among APCs, Th cells, and immunocompetent T and B cells results in a second stage of cellular proliferation and differentiation (more details in Figures 8-19 and 8-22). Because antigen has “selected” those T and B cells with compatible antigen receptors, only a small population of T and B cells undergo this process at one time. The result is an active cellular immunity or humoral immunity, or both. Cellular immunity is mediated by a population of “effector” T cells that can kill targets (cytotoxic T cells) or regulate the immune response (T-regulatory cells), as well as a population of memory cells (memory T cells) that can respond more quickly to a second challenge with the same antigen. Humoral immunity is mediated by a population of soluble proteins (antibodies) produced by plasma cells and by a population of memory B cells that can produce more antibody rapidly to a second challenge with the same antigen.
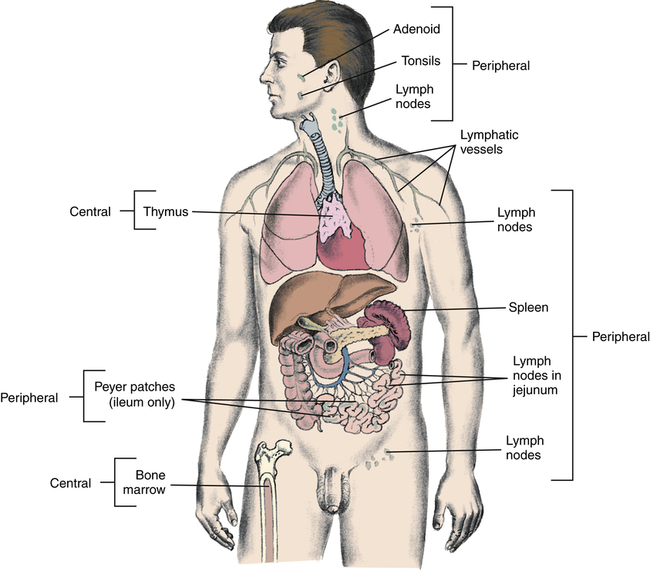
Immature lymphocytes migrate through central (primary) lymphoid tissues: the bone marrow (central lymphoid tissue for B lymphocytes) and the thymus (central lymphoid tissue for T lymphocytes). Mature lymphocytes later reside in the T and B lymphocyte–rich areas of the peripheral (secondary) lymphoid tissues.
Humoral and Cell-Mediated Immunity
The immune response has two arms: antibody and T cells, both of which protect against infection.2 Antibody circulates in the blood and binds to antigens on infectious agents. This interaction can result in direct inactivation of the microorganism or activation of a variety of inflammatory mediators (e.g., complement, phagocytes) that will destroy the pathogen. Antibody is primarily responsible for protection against many bacteria and viruses. This arm of the immune response is termed humoral immunity.
Recognition and Response
The foundation of any successful immune response is the specific recognition of antigen by antibody or receptors on the surface of B or T cells, followed by a set of complex intercellular communications among a variety of antigen-presenting cells and lymphocytes. To fully understand the immune response, it is necessary to initially understand the basis for that recognition. Many of the molecules discussed in this chapter are part of a nomenclature that uses the prefix “CD” followed by a number (e.g., CD1 or CD2) (Table 8-2). The definition of the CD (cluster of differentiation) format has changed over time. It was originally used to describe proteins found on the surface of lymphocytes. Currently, CD is the accepted format for labeling a very large family of proteins found on the surface of many cells. Many have alternative names, which may be used in this chapter. The list of identified molecules is constantly increasing (the number of molecules with a CD designation is probably in excess of 250). In a similar fashion, the list of known cytokines is continually growing, with more than 100 having been identified so far. A large number of CD molecules and cytokines contribute to the acquired immune response. We have attempted to focus on a small number of highly important examples to illustrate the immensely complicated, but highly effective, interactions that take place to produce a protective immune response.
TABLE 8-2
SELECT CD MOLECULES AND THEIR FUNCTIONS
CD MOLECULES | PRIMARY LOCATION | FUNCTIONS |
CD1 | APCs | Presents lipid antigens |
CD2 | All T cells, NK cells | T-cell marker; adhesion molecule that binds to CD58 (LFA-3) and provides a co-stimulatory signal |
CD3 | All T cells | Associated with TCR and provides intracellular signaling |
CD4 | Th cells | Binds to MHC class II as co-receptor with the TCR |
CD8 | Tc cells | Binds to MHC class I as co-receptor with the TCR |
CD19 | B cells | Complexes with CD21 to form a co-receptor for B cells |
CD20 | B cells | Major regulator of B-cell function |
CD21 | B cells | Receptor for complement that complexes with CD19 to form a co-receptor for B cells |
CD25 | Activated T cells | α-Chain of IL-2 receptor |
CD28 | T cells | Adhesion molecule that binds to CD80 to provide co-stimulatory signal for Tc cells |
CD40 | B cells, macrophages | Adhesion molecule that binds to CD154 to provide co-stimulatory signal for B cells |
CD45 | All lymphocytes | Has multiple types; augments antigen signal |
CD58 (LFA-3) | Most cells | Adhesion molecule that binds to CD2 to provide a co-stimulatory signal |
CD80 (B7-1) | APCs | Adhesion molecule that binds to CD28 to provide a co-stimulatory signal |
CD154 (CD40L) | Th2 cells | Adhesion molecule that binds to CD40 to provide a co-stimulatory signal |
Antigens and Immunogens
To function as an antigen, at least a portion of a molecule’s chemical structure must be recognized by and bound to an antibody and/or to specific receptors on a lymphocyte. The precise portion of the antigen that is configured for recognition and binding is called its antigenic determinant, or epitope. The matching portion on the antibody or the lymphocyte receptor is sometimes referred to as the antigen-binding site, or paratope. The size of an antigenic determinant is relatively small, perhaps just a few amino acids or carbohydrate residues on the surface of a large molecule (Figure 8-4). Therefore, macromolecules (e.g., proteins, polysaccharides, nucleic acids) usually contain multiple and diverse antigenic determinants, and the immune response against the macromolecule will usually consist of a mixture of specific antibodies against several of these determinants.
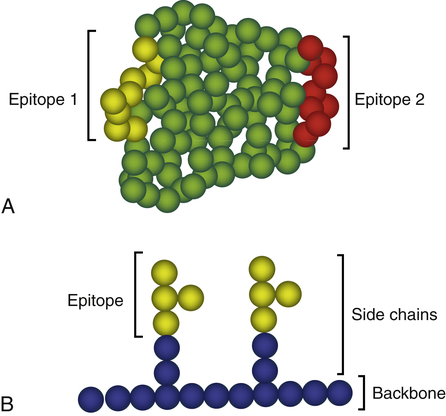
Shown are generic examples of epitopes on protein (A) and polysaccharide (B) molecules. In A, an antigenic protein may have multiple different epitopes (epitopes 1 and 2) that react with different antibodies. Each sphere represents an amino acid with the yellow spheres representing epitope 1 and the red spheres representing epitope 2. Individual epitopes may consist of eight or nine amino acids. In B, a polysaccharide is constructed of a backbone with branched side chains. Each sphere represents an individual carbohydrate with the yellow spheres representing the carbohydrates that form the epitope. In this example, two identical epitopes are shown that would bind two identical antibodies.
Foremost among the criteria for immunogenicity is the antigen’s foreignness. A self-antigen that fulfills all these criteria except foreignness does not normally elicit an immune response. Thus most individuals are tolerant to their own antigens. The immune system has an exquisite ability to distinguish self (self-antigens) from non-self (foreign antigens). Tolerance, once thought to be a state of nonresponsiveness in which the immune system passively allowed self-antigens to persist, is now known to have a variety of mechanisms. In some cases, a state of central tolerance exists, in which lymphocytes with receptors against self-antigens have been eliminated. In other cases, tolerance is peripheral tolerance and part of the adaptive immune response. Rather than merely tolerating some self-antigens, the immune system actively prevents their recognition by lymphocytes and antibodies. The response to self-antigens may be actively regulated by specialized T lymphocytes called T-regulatory (Treg) cells (see Figure 8-2). Some pathogens have a survival advantage by their capacity to mimic self-antigens and avoid inducing an immune response.
Molecules That Recognize Antigen
Antigen is directly recognized by three molecules: circulating antibody and antigen receptors on the surface of B lymphocytes (B-cell receptor, or BCR) and T lymphocytes (T-cell receptor, or TCR) (Figure 8-5).
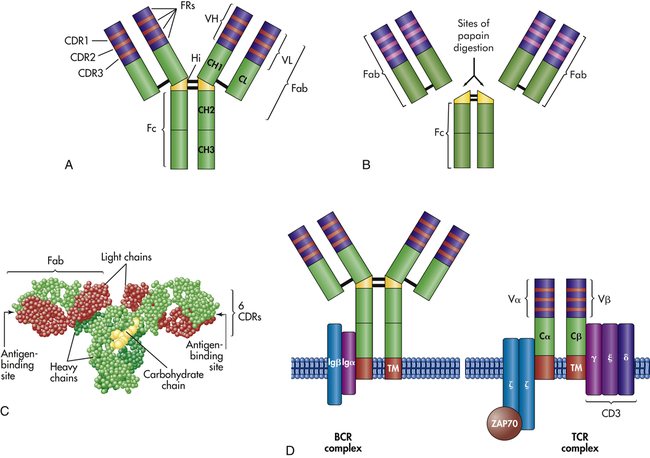
Antigen-binding molecules include soluble antibody (A, B, C) and cell surface receptors (D). A, The typical antibody molecule consists of two identical heavy chains and two identical light chains connected by interchain disulfide bonds (− between chains in the figure). Each heavy chain is divided into three regions with relatively constant amino acid sequences (CH1, CH2, and CH3) and a region with a variable amino acid sequence (VH). Each light chain is divided into a constant region (CL) and a variable region (VL). The hinge region (Hi) provides flexibility in some classes of antibody. Within each variable region are three highly variable complementary-determining regions (CDR1, CDR2, CDR3) separated by relatively constant framework regions (FRs). B, Fragmentation of the antibody molecule by limited digestion with the enzyme papain has identified three important portions of the molecule: an Fc and two identical Fab fragments. Both Fab fragments bind antigen. As the antibody folds (C), the CDRs are placed in proximity to form the antigen-binding site. D, The antigen receptor on the surface of B cells (BCR complex) is a monomeric antibody with a structure similar to that of circulating antibody, with an additional hydrophobic transmembrane region (TM) that anchors the molecule to the cell surface. The active BCR complex contains molecules (Igα and Igβ) that are responsible for intracellular signaling after the receptor has bound antigen. The T-cell receptor (TCR) consists of an α-chain and a β-chain joined by a disulfide bond. Each chain consists of a constant region (Cα and Cβ) and a variable region (Vα and Vβ). Each variable region contains CDRs and FRs in a structure similar to that of antibody. The active TCR is associated with several molecules that are responsible for intracellular signaling. These include CD3, which is a complex of γ (gamma), ε (epsilon), and δ (delta) subunits, and a complex of two ζ (zeta) molecules. The ζ molecules are attached to a cytoplasmic protein kinase (ZAP70) that is critical to intracellular signaling. (C from Patton KT, Thibodeau GA: Anatomy & physiology, ed 8, St Louis, 2012, Mosby.)
Antibody
An antibody, or immunoglobulin, is a serum glycoprotein produced by plasma cells in response to a challenge by an immunogen. The term immunoglobulin is used to denote all molecules that are known to have specificity for antigen, whereas the term antibody is generally used to denote one particular set of immunoglobulins with specificity against a known antigen. There are five molecular classes of immunoglobulins (IgG, IgA, IgM, IgE, and IgD) that are characterized by antigenic, structural, and functional differences (Figure 8-6). Within two of the immunoglobulin classes are several distinct subclasses including four subclasses of IgG and two subclasses of IgA.
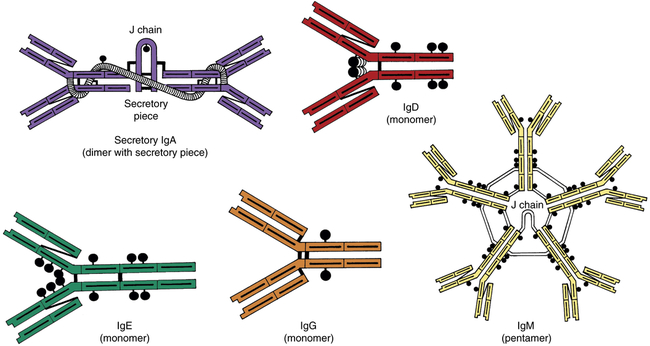
Secretory IgA, IgD, IgE, IgG, and IgM. The black circles attached to each molecule represent carbohydrate residues.
Classes
IgG is the most abundant class of immunoglobulins; they constitute 80% to 85% of those circulating in the body and account for most of the protective activity against infections (Tables 8-3 and 8-4). As a result of selective transport across the placenta, maternal IgG is also the major class of antibody found in blood of the fetus and newborn. Four subclasses of IgG have been described: IgG1, IgG2, IgG3, and IgG4.
TABLE 8-3
PHYSICOCHEMICAL PROPERTIES OF IMMUNOGLOBULINS
CLASS | SUBCLASS | HEAVY CHAIN | MOLECULAR WEIGHT (DALTONS) | ADULT SERUM LEVELS (mg/dl) |
IgG | IgG1 | (γ1) | 146,000 | 800-900 |
IgG2 | (γ2) | 146,000 | 280-300 | |
IgG3 | (γ3) | 165,000 | 90-100 | |
IgG4 | (γ4) | 146,000 | 50 | |
IgM | IgM | (μ) | 970,000 | 120-150 |
IgA | IgA1 | (α1) | 160,000 | 280-300 |
IgA2 | (α2) | 50 | ||
sIgA | (α1, α2) | 385,000 | 5 | |
IgD | IgD | (δ) | 184,000 | 3 |
IgE | IgE | (ε) | 190,000 | 0.03 |
TABLE 8-4
BIOLOGIC PROPERTIES OF IMMUNOGLOBULINS
SUBCLASS | COMPLEMENT ACTIVATION | BINDING TO FC RECEPTORS ON | PLACENTAL TRANSFER | PRESENCE IN SECRETIONS | INDUCTION OF AGGLUTINATION | ||||
CLASSICAL | ALTERNATIVE | MACROPHAGES | PMNs | MAST CELLS | PLATELETS | ||||
IgG1 | ++ | − | + | + | − | + | +++ | ± | + |
IgG2 | + | − | − | − | − | + | + | ± | + |
IgG3 | +++ | − | + | + | − | + | +++ | ± | + |
IgG4 | − | − | − | ± | + | + | ++ | ± | |
IgM | ++++ | − | − | − | − | − | − | + | +++ |
IgA1 | − | + | − | ± | − | − | − | + | − |
IgA2 | − | + | − | ± | − | − | − | + | − |
sIgA | − | − | − | − | − | − | − | ++++ | − |
IgD | − | ± | − | − | − | − | − | − | − |
IgE | − | ± | ? | − | +++ | − | − | + | − |
IgE is the least concentrated of any of the immunoglobulin classes in the circulation. It appears to have very specialized functions as a mediator of many common allergic responses (see Chapter 9) and in the defense against parasitic infections.
Molecular Structure
Structural analysis of immunoglobulins began with Porter’s early studies on the effects of the enzyme papain on IgG.3 The nomenclature of antibody structure originated from that work. Limited digestion with the enzyme papain cleaved IgG into three fragments, two of which were identical. The two identical fragments were found to retain the ability to bind antigen, and each was termed an antigen-binding fragment (Fab).4 The third fragment crystallized when separated from the Fab portions and was termed the crystalline fragment (Fc) (see Figure 8-5).
The basic structure of the antibody molecule consists of four polypeptide chains—two identical light (L) chains and two identical heavy (H) chains (see Figure 8-5). Within the same molecule, the two heavy chains are identical and the two light chains are identical. The class of antibody is determined by which heavy chain is used: gamma (IgG), mu (IgM), alpha (IgA), epsilon (IgE), or delta (IgD). The light chains of an antibody molecule are of either the kappa (κ) or the lambda (λ) type. The light and heavy chains are held together by two major forces: noncovalent bonds and disulfide linkages. A set of disulfide linkages between the heavy chains occurs in the hinge region and in some instances lends a degree of molecular flexibility at that site so that the Fab regions can move.
Antigen Binding
The combined amino acid sequences of the variable regions of both the heavy (VH) and light (VL) chains determine the conformation of the antigen-binding site and therefore the antigenic specificity of the immunoglobulin molecule. Most proteins will naturally fold and take on secondary or tertiary structures. As the immunoglobulin molecules fold, the FRs control the accuracy of folding in the variable region, and the CDRs in both variable regions are moved into proximity, resulting in an antigen-binding site formed by the three CDRs of the heavy chain and the three CDRs of the light chain. The chemical nature of the particular amino acids in those sites, as well as the topography of the site, determines the specificity toward a particular antigen. The antigen that will bind most strongly must have complementary chemistry and topography with the binding site formed by the antibody. The antigen fits into this binding site with the specificity of a key into a lock and is held there by noncovalent chemical interactions (Figure 8-7). In some cases the substitution of a single critical amino acid in a CDR may have a significant effect on the shape of the binding site and thus the specificity of the antibody molecule.
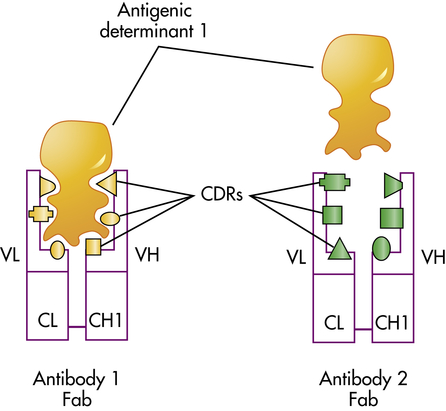
The specificity required for antibody binding with an antigen is determined by the shape and chemistry of the six complementary-determining regions (CDRs) in the combining site on the variable region of the antibody. This figure indicates two different antibodies (Fab portions of antibody 1 and antibody 2) that have different sets of CDRs and therefore different specificities. As indicated, the antigenic determinant that reacts well with antibody 1 is unable to react with antibody 2 because of differences in the antibody combining site. C, Constant; CH, constant region of the heavy chain; CL, constant region of the light chain; Fab, antigen-binding fragment, V, variable; VH, variable heavy chain, VL, variable light chain.
B-Cell Receptor Complex
The B-cell receptor (BCR) complex is located on the surface of B lymphocytes (see Figure 8-5). Its role is to recognize antigen, but unlike circulating antibody, the receptor must communicate that information to the cell’s nucleus. Therefore, the BCR complex consists of antigen-recognition molecules and accessory molecules involved in intracellular signaling (Igα and Igβ). BCRs on the surface of immunocompetent B cells are membrane-associated IgM (mIgM) and IgD (mIgD) immunoglobulins that are produced from the same genes that are used by plasma cells to produce soluble antibodies. As a BCR, however, mIgM is a monomer rather than the pentamer primarily found in the blood.
T-Cell Receptor Complex
T lymphocytes use a similar but distinct array of proteins in their recognition and response to antigens. The T-cell receptor (TCR) complex is composed of an antibody-like transmembrane protein (TCR) and a group of accessory proteins (collectively referred to as CD3) that are involved in intracellular signaling (see Figure 8-5). Similar to activation of the B lymphocyte, the TCR is responsible for recognition and binding to the antigen, whereas the accessory proteins are responsible for the intracellular signaling necessary for activation and differentiation of the T cell. Each of the individual components of the TCR complex is important, and several severe defects in the T-cell immune response have been related to mutations in individual components of the complex (see Chapter 9).
Molecules That Present Antigen
Major Histocompatibility Complex
MHC molecules are glycoproteins found on the surface of all human cells except red blood cells. They are divided into two general classes, class I and class II, based on their molecular structure, distribution among cell populations, and function in antigen presentation. MHC class I molecules are heterodimers composed of a large α-chain along with a smaller chain called β2-microglobulin. MHC class II molecules are also heterodimers with both α- and β-chains. The general properties of each of the MHC classes are summarized in Figure 8-8.
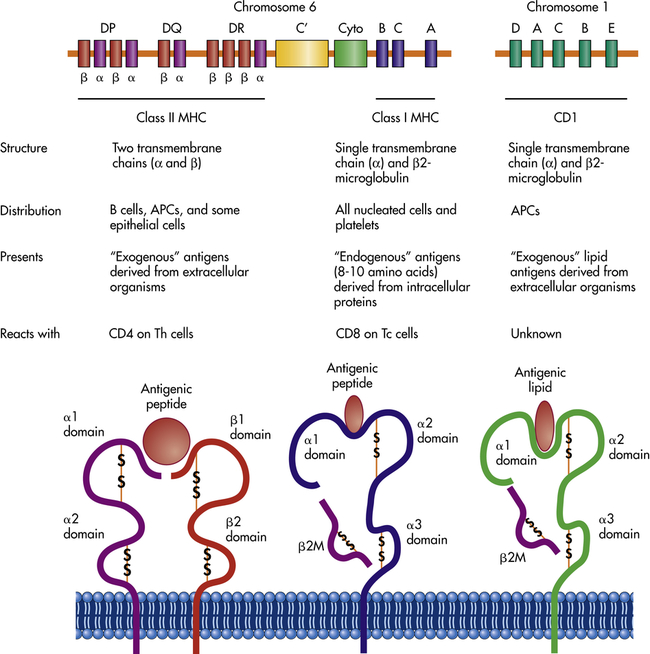
Three sets of molecules are primarily responsible for antigen presentation: MHC class I, MHC class II, and CD1. The MHC molecules are encoded from the MHC region on chromosome 6, which contains information for class I and class II molecules, as well as for several other molecules that participate in the innate or immune responses. These include several complement proteins (C′) and cytokines (Cyto), which are referred to as MHC class III molecules. Three principal class I molecules, HLA-A, HLA-B, and HLA-C, are presented here, but this region contains information for the α-chains of several other molecules, including HLA-E, HLA-F, and HLA-G. The MHC class I products complex with β2-microglobulin, which is encoded by a gene on chromosome 15. The MHC class I molecules present small peptide antigens in a pocket formed by the α1 and α2 domains of the α-chain. The conformation of the molecule is stabilized by β2-microglobulin (β2M) as well as by intrachain disulfide bonds (-S-S-). The α- and β-chains of class II molecules are also encoded in this region: HLA-DR, HLA-DP, and HLA-DQ. In some cases, multiple genes for α- and β-chains are available. The MHC class II molecules present peptide antigens in a pocket formed by the α1 domain of the α-chain and the β1 domain of the β-chain. The genes for CD1 molecules are encoded on chromosome 1, which contains genes for five α-chains (CD1A-E), and the α-chains complex with β2-microglobulin to present lipid antigens in a pocket formed by the α1 and α2 domains. All three sets of antigen-presenting molecules are anchored to the plasma membrane by hydrophobic regions on the ends of the α- and β-chains. MHC, Major histocompatibility complex.
Molecules of the two MHC classes are encoded from different genetic loci that are located as a large complex of genes on the short arm of human chromosome 6 (see Figure 8-8). The MHC also contains other genes that control the quality and quantity of an immune response, which are commonly referred to as class III MHC genes. The primary MHC class I genes consist of three closely linked loci on this chromosome labeled A, B, and C. The primary MHC class II genes are located within the D region, which actually consists of three separate and independent loci: DR, DP, and DQ.
Transplantation
The specific combination of alleles at the six major HLA loci on one chromosome (A, B, C, DR, DQ, and DP) is termed a haplotype. Each individual has two HLA haplotypes, one from the paternal chromosome 6 and another from the maternal chromosome. Because the different HLA loci within the MHC are in such close proximity to one another, haplotypes are not usually disrupted by recombination and are thus inherited intact. Each parent passes on one HLA haplotype to each of his or her offspring, meaning that children usually share one haplotype with each parent (Figure 8-9). Odds dictate that children will share one haplotype with half of their siblings and either no haplotypes or both haplotypes with a quarter of their siblings. Thus the chance of finding a match among siblings is much higher (25%) than that from the general population.
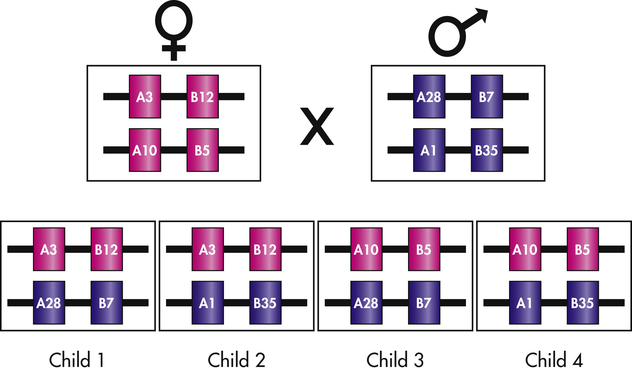
HLA alleles are inherited in a codominant fashion so that both maternal and paternal antigens are expressed. Specific HLA alleles are commonly given numbers to indicate different antigens. In this example, the mother has linked genes for HLA-A3 and HLA-B12 on one chromosome 6 and genes for HLA-A10 and HLA-B5 on the second chromosome 6. The father has HLA-A28 and HLA-B7 on one chromosome and HLA-A1 and HLA-B35 on the second chromosome. On one particular chromosome, the HLA antigens are firmly linked, with crossovers occurring in only 1% of individuals. The children from this pairing may have one of four possible combinations of maternal and paternal HLA. HLA, Human leukocyte antigen.
Molecules That Hold Cells Together
The efficient development of an immune response requires several antigen-independent interactions between cells. The interactions between specific cellular receptors and their ligands result in intracellular signaling events that are independent of the TCR or BCR complexes but are necessary complements to the antigen-specific signal. Several of these molecules are listed in Box 8-1.
Cytokines and Their Receptors
As discussed in Chapter 7, cytokines are low-molecular-weight proteins, or glycoproteins, that function as chemical signals between cells. A large number of cytokines are secreted by APCs and lymphocytes and provide both positive and negative regulation of the immune response. The effects of particular cytokines depend on binding to specific cellular receptors, which are linked to intracellular signaling pathways. The lymphocyte may respond in many ways. One of the most common responses is an increase in the production of proteins, many of which are other cytokines or cytokine receptors. Many cytokines also cause a lymphocyte to proliferate and differentiate. The participation of cytokines is essential to the development of an adequate immune response, and in general, the precise combination of cytokines influences the ultimate response of a given cell. Specific deficiencies in the immune response that result from genetic mutations that lead to defective cytokine production or defective cytokine receptors are discussed in Chapter 9. Table 8-5 provides information about key cytokines and receptors that are known to influence the immune response.
TABLE 8-5
KEY CYTOKINES AND RECEPTORS THAT INFLUENCE THE IMMUNE RESPONSE
CYTOKINE | PRIMARY SOURCE | PRIMARY FUNCTION |
Interleukin (IL) | ||
IL-1 | APCs | Stimulates T cells to proliferation and differentiation; induces acute-phase proteins in inflammatory response; endogenous pyrogen |
IL-2 | Th1 cells, NK cells | Stimulates proliferation and differentiation of T cells and NK cells |
IL-4 | Th2 cells, mast cells | Induces B-cell proliferation and differentiation; up-regulates MHC class II expression; induces class-switch to IgE |
IL-5 | Th2 cells, mast cells | Induces eosinophil proliferation and differentiation; induces B-cell proliferation and differentiation |
IL-6 | Th2 cells, APCs | Induces B-cell proliferation and differentiation into plasma cells; induces acute-phase proteins in inflammatory response |
IL-7 | Thymic epithelial cells, bone marrow stromal cells | Major cytokine for induction of B- and T-cell proliferation and differentiation in central lymphoid organs |
IL-8 | Macrophages | Chemotactic factor for neutrophils |
IL-10 | Th cells, B cells | Inhibits cytokine production; activator of B cells |
IL-12 | B cells, APCs | Induces NK-cell proliferation; increases production of IFN-γ |
IL-13 | Th2 cells | IL-4–like properties; decreases inflammatory responses |
IL-17 | Th17 cells | Increases inflammation; increased influx of neutrophils and macrophages; increased epithelial cell chemokine production |
IL-22 | Th17 cells | Increases inflammation; increased epithelial cell production of antimicrobial peptides |
Interferon (IFN) | ||
IFN-α, IFN-β | Macrophages, some virally infected cells | Antiviral; increases expression of MHC class I; activates NK cells |
IFN-γ | Th1 cells, NK cells, Tc cells | Increases expression of MHC class II; activates macrophages and NK cells |
Tumor Necrosis Factor (TNF) | ||
TNF-α (cachectin) | Macrophages | IL-1–like properties; induces cellular proliferation |
TNF-β (lymphotoxin) | Tc cells | Kills some cells; increases phagocytosis by macrophages and neutrophils |
Transforming Growth Factor (TGF) | ||
TGF-β | Lymphocytes, macrophages, fibroblasts | Chemotactic for macrophages; increases macrophage IL-1 production; stimulates wound healing |
CYTOKINE RECEPTORS | LIGAND | ADDITIONAL INFORMATION |
Class I receptor dimers (α- and β-chains) | IL-3, IL-5, IL-6, IL-11, IL-12, IL-13 | IL-3 and IL-5 share a common α-chain; IL-6 and IL-11 share a common β-chain |
Trimers (α-, β-, and γ-chains) | IL-2, IL-4, IL-7, IL-9, IL-15 | All share a common γ-chain |
Class II receptors | IFN-α, -β, and -γ | Two chains |
TNF receptors | TNF-α, TNF-β, CD40, Fas | Single chain |
Immunoglobulin-like receptors | IL-1 | Single chain with immunoglobulin-like characteristics |
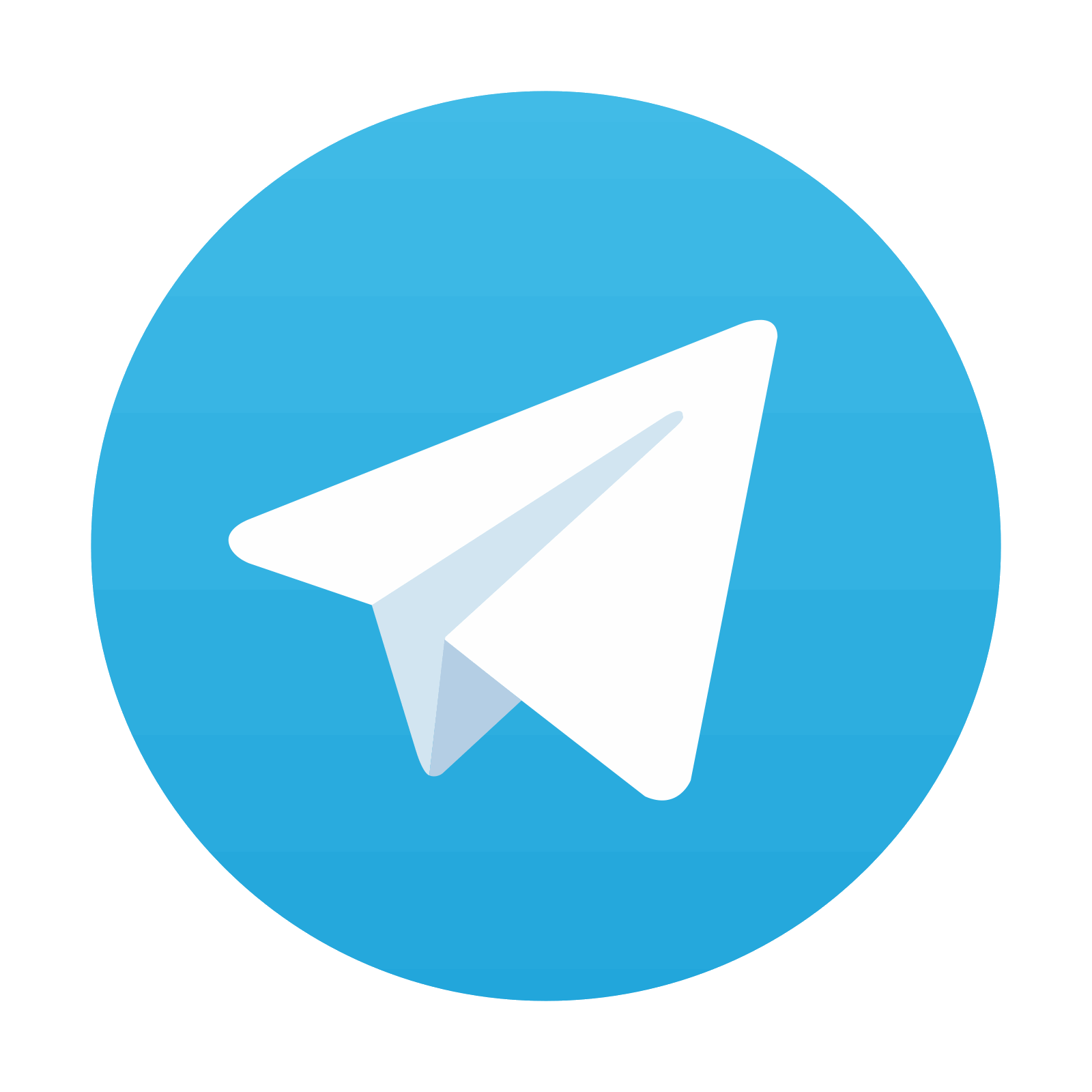
Stay updated, free articles. Join our Telegram channel
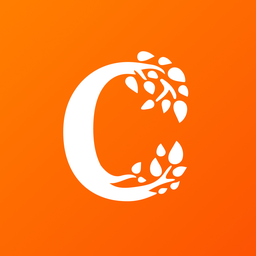
Full access? Get Clinical Tree
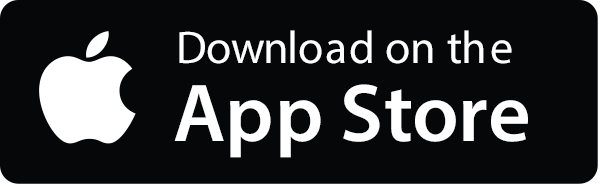
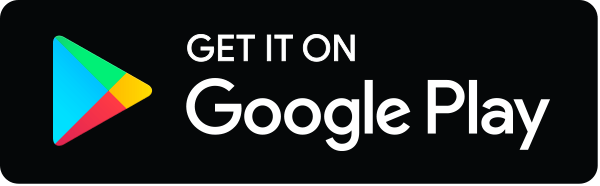