PARASITOLOGY
DEFINITION
The plasmodia are Apicomplexa in which the sexual and asexual cycles of reproduction are completed in different host species. The sexual phase occurs within the gut of mosquitoes and results in the formation of a motile zygote, the ookinete. These arthropods subsequently transmit the parasite as sporozoites while feeding on a vertebrate host. Within the vertebrate, the plasmodia reproduce asexually, first in the liver and then in erythrocytes; they eventually burst from the erythrocyte and invade other uninvolved RBCs. This event produces periodic fever and anemia in the host, a disease process known as malaria. Of the many species of plasmodia, five are known to infect humans and are considered here: Plasmodium vivax, P ovale, P malariae, P knowlesi, and P falciparum. The general apicomplexan cell plan is illustrated in Figure 51–1.
FIGURE 51–1. The apicomplexan cell. (Reproduced with permission from Willey JM: Prescott, Harley, & Klein’s Microbiology, 7th edition. McGraw-Hill, 2008.)
Sexual phase in mosquito and asexual phase in humans
Five species infect humans
LIFE CYCLE OF MALARIAL PARASITES
This day relenting God
Hath placed within my hand
A wondrous thing; and God
Be praised. At his command,
With tears and toiling breath,
I find thy cunning seeds,
O million-murdering Death.
I know this little thing
A myriad men will save,
O Death, where is thy sting?
Thy victory, O Grave?
—Sir Ronald Ross, August 22, 1897, in a poem to Sir Patrick Manson on the discovery of sporo-zoites in mosquito salivary glands.
The life cycle in the female Anopheles mosquito begins with the ingestion of male and female gametocytes from the circulation of a malaria-infected individual. In the gut of the mosquito, the gametocytes mature, are released from infected erythrocytes, and effect fertilization. The resulting zygote, an ookinete, is the only stage in the life cycle that is diploid, is motile, and penetrates the mosquito’s gut wall, lodges beneath the basement membrane facing the mosquito’s hemocoel, undergoes a postzygotic reduction division, and vacu-olates to form an oocyst. Within this structure, thousands of sporozoites are formed by asexual division. The enlarging cyst eventually ruptures, releasing the sporozoites into the body cavity of the mosquito. Some penetrate the salivary glands, rendering the mosquito infectious for humans. The time required for the completion of the cycle in mosquitoes varies from 1 to 3 weeks, depending on the species of insect and parasite as well as on the ambient temperature and humidity.
Mosquito ingests gametocytes from blood of infected human
Sporozoites from oocyst reach mosquito salivary glands
Sporozoites from the mosquito’s salivary glands are injected into the human’s subcutaneous capillaries when the female mosquito feeds. Within minutes and up to 1 hour they attach to and invade liver cells (hepatocytes), a process mediated by a ligand present in the outer protein coat of the sporozoites (circumsporozoite protein). In P vivax and P ovale infections, some of the sporozoites enter a dormant state immediately after cell invasion to become hypnozoites. These stages are responsible for the relapse phenomenon seen in malarial infections caused by these species. In all malarial infections, the remaining sporozoites initiate exoerythrocytic schizogony, each producing about 2000 to 40 000 daughter cells, or merozoites, depending on the infecting species. After 1 to 2 weeks, the infected hepatocytes rupture, releasing merozoites into the general circulation.
Humans infected by mosquito bite
Rapid infection of hepatocytes starts asexual cycle in humans
The erythrocytic phase of malaria starts with the attachment of a released hepatic merozoite to a specific receptor on the RBC surface. After attachment, the merozoite releases substances from its apical organelles, the rhoptries, which affects red cell membrane fluidity resulting in invagination of the cell membrane and entry of the parasite into a parasitophorous vacuole. The intracellular parasite initially appears as a small ring-shaped trophozoite, which enlarges and becomes more active and irregular in outline. Within a few hours, nuclear division occurs, producing the multinucleated schizont. The cytoplasm eventually condenses around each nucleus of the schizont to form an intraerythrocytic cluster of 6 to 24 merozoite daughter cells. About 24 (P knowlesi), 48 (P vivax, P ovale, and P falciparum) to 72 (P malariae) hours after initial invasion, infected erythrocytes rupture, releasing the merozoites and producing the first clinical manifestations of disease. The newly released merozoites invade other RBCs, where most repeat the asexual cycle. Other merozoites are transformed into sexual forms or gametocytes. These latter forms do not produce RBC lysis and continue to circulate in the peripheral vasculature until ingested by an appropriate mosquito. The recurring asexual cycles continue, involving an ever-increasing number of erythrocytes until the development of host immunity helps contain the erythrocytic cycle. The dormant hepatic sporozoites of P vivax and P ovale survive the host’s immunologic attack and may, after a latent period of months to years, resume intrahepatic multiplication. This leads to a second release of hepatic merozoites and the initiation of another erythrocytic cycle, a phenomenon known as relapse. The life cycle of malarial parasites is summarized in Figure 51–2 and variations in the differential characteristics of the parasites infecting humans are summarized in Table 51–1.
FIGURE 51–2. Drawings of erythrocytic stages of malarial parasites. Note that trophozoite and schizont forms of Plasmodium falciparum occur in visceral capillaries rather than in blood. Female gametophytes have morphologic differences from the male forms shown. (Reproduced with permission from Connor DH, Chandler FW, Schwartz DQ, et al: Pathology of Infectious Diseases. Stamford CT: Appleton & Lange, 1997.)
TABLE 51–1 Differential characteristics of Plasmodium Species
Erythrocytic cycle begins with merozoite attachment to RBC receptor
Trophozoites multiply in RBCs to form new merozoites
In 48 to 72 hours, RBcs rupture, releasing merozoites to infect new RBcs
Intrahepatic dormancy causes relapses with P vivax and P ovale
MORPHOLOGY OF ERYTHROCYTIC PARASITES
The morphology of the stained intraerythrocytic Plasmodium parasites is shown in Figure 51–3. In stained smears, three characteristic features aid in the identification of plasmodia: Red nuclear chromatin; blue cytoplasm; and brownish-black malarial pigment, or hemozoin, consisting largely of a hemoglobin degradation product, ferriprotoporphyrin IX. The change in the shape of the cytoplasm and the division of the chromatin at different stages of parasite development are obvious. Gametocytes can be differentiated from the asexual forms by their large size and lack of nuclear division. Some of the infected erythrocytes develop membrane invaginations or caveolae-vesicle complexes, which are thought to be responsible for the appearance of the pink Schüffner dots or granules (see following text).
FIGURE 51–3. Malaria. Life cycle of Plasmodium vivax. (Reproduced with permission from Willey JM: Prescott, Harley, & Klein’s Microbiology, 7th edition. McGraw-Hill, 2008.)
Morphology of the parasite and the infected RBCs vary by stage and species
The appearance of each of the five species of plasmodia that infect humans is sufficiently different to allow their differentiation in stained smears, although some similarities in some stages exist between the different species. The parasitized erythrocyte in P vivax and P ovale infections is pale and enlarged and contains numerous Schüffner dots. All asexual stages (trophozoite, schizont, merozoite) may be seen simultaneously. Cells infected by P ovale are elongated and frequently irregular or fimbriated in appearance. In P malariae infections, the RBCs are not enlarged and contain no granules. The trophozoites often present as “band” forms, and the merozoites are arranged in rosettes around a clump of central pigment. In P falciparum infections, the rings are very small and may contain two chromatin dots rather than one. There is often more than one parasite per cell, and parasites are frequently seen lying against the margin of the cell. Intracytoplasmic granules known as Maurer dots may be present, but are often cleft shaped and fewer in number than Schüffner dots. Schizonts and merozoites are not present in the peripheral blood as they are sequestered in postcapil-lary venules. Gametocytes are large and banana shaped. Plasmodium knowlesi shares many of the morphologic characteristics of P malariae, but can be distinguished from the latter by its fever cycle and diagnostically by using polymerase chain reaction (PCR). These characteristics are summarized in Table 51–2.
TABLE 51–2 Chemotherapy of Malaria
Morphologic differences are the primary means of diagnosis
PHYSIOLOGY
Species of plasmodia differ significantly in their ability to invade subpopulations of eryth-rocytes; P vivax and P ovale attack only immature cells (reticulocytes), whereas P malariae attacks only senescent cells. During infection with these species, therefore, no more than 1% to 2% of the cell population is involved. Plasmodium falciparum, in contrast, invades RBCs, regardless of age, and may produce very high levels of parasitemia and particularly serious disease. In part, these differences may be related to the known differences in the RBC receptor sites available to the individual Plasmodium species. In the case of P vivax, the site is closely related to the Duffy blood group antigens (Fya and Fyb). Duffy-negative individuals, who constitute the majority of people of West African ancestry, are therefore resistant to vivax malaria. RBC sialoglycoproteins, particularly glycoprotein A, has been implicated as the P falciparum receptor site.
Parasites vary in ability to attack subpopulations of erythrocytes
RBc Duffy antigen and glycoprotein A are RBc receptors
Certain RBC abnormalities may also affect parasitism. The altered hemoglobin (hemoglobin S) associated with the sickle cell trait limits the intensity of the parasitemia caused by P falciparum, and thereby provides a selective advantage to individuals who are heterozygous for the sickle cell gene. As a result, the sickle cell gene, which would otherwise be disadvantageous, is very common in populations living in malarious areas. Parasite growth appears to be retarded in RBCs heterozygous for hemoglobin S (SA) when they are exposed to conditions of reduced oxygen tension such as those which might be present in the visceral capillaries. These conditions cause the hemoglobin in infected cells to polymerize, rendering it unusable by the parasite. In essence, the parasite starves to death. Sickling may also render the erythrocyte more susceptible to phagocytosis or directly damage the parasite. A similar protective effect may be exerted by hemoglobins C, D, and E; thalassemias; and glucose-6-phosphate dehydrogenase (G6PD) or pyridoxal kinase deficiencies, because these abnormalities have also been found more frequently in malarious areas. The protection in these conditions may be related to the increased susceptibility of such RBCs to oxidant stress. In thalassemia, the protection may also be related in part to the production of fetal hemoglobin, which retards maturation of P falciparum, as well as an increased binding of antibodies to modified parasitic antigens (neoantigens) presenting on the surface of the erythrocytes.
Sickle cell trait limits intensity of P falciparum infection
Other hemoglobinopathies can also exert protection
Once invasion has occurred, malaria parasites may induce a number of changes in the erythrocytic membrane. These include alteration of its lipid concentration, modification of its osmotic properties, and incorporation of parasitic neoantigens, rendering the RBCs susceptible to immunologic attack. Plasmodium vivax and P ovale stimulate the production of caveolae-vesicle complexes, which are visualized as Schüffner dots in stained smears. In P fal-ciparum infections, electron-dense elevated knobs or excrescences form on the RBC surface. These produce a strain-specific, high-molecular-weight adhesive protein (PfEMP1), which mediates binding to receptors on the endothelium of capillaries and postcapillary venules of the brain, placenta, and other organs, where they can produce obstruction and microinfarcts.
Changes induced in erythrocyte membrane
Binding to endothelium may cause microinfarcts
Malarial parasites generate energy by the anaerobic metabolism of glucose. They appear to satisfy their protein requirements by the degradation of hemoglobin within their acidic food vacuoles, resulting in the formation of the malarial pigment (hemozoin) mentioned previously. It has been estimated that the average plasmodium destroys between 25% and 75% of the hemoglobin of its host erythrocyte. Unlike their vertebrate hosts, malarial parasites synthesize folates de novo. As a result, antifolate antimicrobials such as pyrimethamine are effective antimalarial agents.
Malarial parasites metabolize anaerobically, synthesize their own folate
GROWTH IN THE LABORATORY
Continuous in vitro cultivation of plasmodia in human erythrocytes was first achieved in 1976. More recently, the successful in vitro completion of the entire sporogonic cycle, from ookinete to sporozoite, has been achieved. These twin developments provide new opportunities for studying the biology, immunology, and chemotherapy of human malaria. The most immediate impact of these advances has been on the introduction of methods for testing the sensitivity of P falciparum to chemotherapeutic agents. Ultimately, these agents will play critical roles in the development of effective antimalarial vaccines.
MALARIA
EPIDEMIOLOGY
Malaria has a worldwide distribution between 45°N and 40°S latitude, generally at altitudes below 1800 m. Plasmodium vivax is the most widely distributed of the four species, and together with the uncommon P malariae, is found primarily in temperate and subtropical areas. Plasmodium falciparum is the dominant organism of the tropics. Plasmodium ovale is rare and found principally in Africa. Plasmodium knowlesi, first recognized in humans in 1965, accounts for up to 70% of the infections recorded in some areas of Southeast Asia. It is also a zoonotic species, causing malaria in long-tailed macaques.
Distribution in tropical areas worldwide
The intensity of malarial transmission in an endemic area depends on the density and feeding habits of suitable mosquito vectors and the prevalence of infected humans, who serve as parasite reservoirs. In hyperendemic areas (areas where more than half of the population is parasitemic), transmission is usually constant, and disease manifestations are moderated by the development of immunity. Mortality is largely restricted to infants and to nonimmune adults who migrate into the region and is primarily caused by P falciparum. When the prevalence of disease is lower, transmission is typically intermittent. In this situation, solid immunity does not develop and the population suffers repeated, often seasonal, epidemics, the impact of which is shared by people of all ages.
Clinical manifestations muted with hyperendemicity
Presently, an estimated 2 billion people live in malaria-endemic areas in 103 of the poorest countries of Africa, Asia, Latin America, and Oceania (Figure 51–4). Between 25% and 50% of these persons are thought to be carrying the malaria parasite at any given time. Approximately 1 million individuals, primarily African children, die of malaria annually. A recent study concluded that the development of resistance to chloroquine, the single most widely used antimalarial agent, has increased mortality four- to eightfold. Although endemic malaria disappeared from the United States three decades ago, imported cases continue to be reported, and the recent worldwide resurgence of malaria combined with an increase in international travel has resulted in an increase in the number of US cases to approximately 1000 annually as reported by the CDC. Forty-five percent of the patients with imported malaria have acquired the disease in Africa, 30% in Asia, and 10% in the Caribbean or Latin America. Fifty percent of recent infections have involved American travelers: Nearly 60% of these acquired their infection in Africa.
FIGURE 51–4. Geographic distribution of malaria. (Reproduced with permission from Willey J, Sherwood L, Woolverton C (eds). Prescott’s Principles of Microbiology. New York: McGraw-Hill; 2008; Data from World Health Statistics Quarterly, 41:69;1988.)
Clinical manifestations of malaria typically develop within 6 months of arrival of cases in the United States; however, 25% of cases caused by P vivax are delayed beyond that time. Approximately 40% of imported cases and almost all associated fatalities have been caused by the virulent P falciparum. Tragically, most of these cases could have been prevented or successfully treated. Congenital malaria in infants born in the United States of mothers from malarious areas is occasionally observed. Infections transmitted by transfusions of whole blood, leukocytes, or platelets, or by organ transplantation are, fortunately, now unusual in this country due to the improved screening procedures of blood banks.
Malaria kills 1 to 3 million annually; mostly children
Imported malaria may develop months after travel
Anopheline mosquitoes capable of transmitting malaria are present throughout much of the United States. On rare occasions, malaria is transmitted from an imported case to individuals who have never traveled outside of the country.
PATHOGENESIS
The fever, anemia, circulatory changes, and immunopathologic phenomena characteristic of malaria are all the result of the erythrocytic cycle of the plasmodia. There are no clinical signs of infection associated with the liver phase of infection.
Fever
Fever, the hallmark of malaria, appears to be initiated by the process of RBC rupture that leads to the liberation of a new generation of merozoites. To date, all attempts to detect the factor(s) mediating the fever have been unsuccessful. It is possible that parasite-derived pyrogens are released at the time of red cell rupture; alternatively, the fever might result from the release of interleukin-1 (IL-1) and/or tumor necrosis factor (TNF) from mac-rophages involved in the ingestion of parasitic or erythrocytic debris. Early in malaria, RBCs appear to be infected with malarial parasites at several different stages of development, each inducing erythrocyte destruction at a different time. The resulting fever is irregular and hectic. Because temperatures higher than 40°C destroy mature parasites, a single population eventually emerges, parasite replication is synchronized, and fever occurs in distinct paroxysms at 24 hour (P knowlesi), 48 hour (P falciparum, P vivax, P ovale) or, in the case of P malariae, 72 hour intervals. Periodicity is seldom seen in patients who are rapidly diagnosed and treated. Periodicity is also not always a hallmark of P falciparum infections. Fever-induced modifications to membrane architecture and infected-cell sequestration events are thought to play a role in disrupting periodicity in these infections. Sometimes, the fever can more or less be continuous.
Fever associated with RBc rupture
Synchronization of parasite replication causes cyclic fever
Anemia
Parasitized erythrocytes are phagocytosed by a stimulated reticuloendothelial system or are destroyed at the time of parasite-induced cell rupture, releasing toxic products. This not only results in destruction of infected cells, but noninfected ones as well, resulting in an anemia that may be disproportionate to the degree of parasitism. Depression of marrow function, sequestration of erythrocytes within the enlarging spleen, and accelerated clearance of nonparasitized cells all appear to contribute to the anemia. So too might cytokine imbalances brought about by overstimulation of innate immune responses. Such imbalances can influence erythropoiesis. Intravascular hemolysis, though uncommon, may occur, particularly in P falciparum malaria. When hemolysis is massive, hemoglobinuria develops, resulting in the production of dark urine. This process in conjunction with malaria is known as blackwater fever.
Destruction of normal and parasitized RBcs causes anemia
Massive intravascular hemolysis can occur
Circulatory Changes
The high fever results in significant vasodilatation. In falciparum malaria, vasodilatation leads to a decrease in the effective circulating blood volume and hypotension, which may be aggravated by other changes in the small vessels and capillaries. The intense parasitemias of P falciparum is capable of producing comas and the adhesion of infected RBCs to the endothelium of visceral capillaries can impair the microcirculation and precipitate tissue hypoxia, lactic acidosis, and hypoglycemia. Although all deep tissues are involved, the brain is the most intensely affected resulting in what has been described as cerebral malaria (Figure 51–5).
FIGURE 51–5. Central nervous system malaria. This small cerebral blood vessel is blocked with many parasitized erythrocytes adherent to the endothelium. (Reproduced with permission from Connor DH, Chandler FW, Schwartz DQ, et al: Pathology of Infectious Diseases. Stamford CT: Appleton & Lange, 1997.)
Blood flow decreased to vital organs
Cytokines
Elevated levels of IL-1 and TNF are consistently found in patients with malaria. Probably released at the time of parasite rupture from erythrocytes, these proteins are certainly an essential part of the host’s immune response to malaria. By modulating the effects of endothelial cells, macrophages, monocytes, and neutrophils, they may play an important role in the destruction of the invading parasite. However, TNF levels increase with parasite density, and high concentrations appear harmful. TNF has been shown to cause upregulation of endothelial adhesion molecules; high concentrations might precipitate cerebral malaria by increasing the sequestration of P falciparum-parasitized erythrocytes in the cerebral vascular endothelium. Alternatively, excessive TNF levels might precipitate cerebral malaria by directly inducing hypoglycemia and lactic acidosis.
Elevated cytokine levels contribute to injury
Other Pathogenic Phenomena
Thrombocytopenia is common in malaria and appears to be related to both splenic pooling and a shortened platelet lifespan. Both direct parasitic invasion and immune mechanisms may be responsible. There may be an acute transient glomerulonephritis in falciparum malaria and progressive renal disease in chronic P malariae malaria. These phenomena probably result from the host immune response, with deposition of immune complexes in the glomeruli.
Thrombocytopenia and nephritis common
IMMUNITY
Once infected, the host quickly mounts a stage-, species-, and strain-specific immunologic response that typically limits parasite multiplication and moderates the clinical manifestations of disease, without eliminating the infection. A prolonged recovery period marked by recurrent exacerbations in both symptoms and number of erythrocytic parasites follows. Recrudescences are marked by periods in which the parasitemia drops below the threshold of detection, only to surge again. Fluctuations in immunity probably account for this phenomenon. With time, these recrudescences become less severe and less frequent, and eventually may stop altogether.
Initial immune response limits parasite multiplication, but does not eliminate infection
The exact mechanisms involved in this recovery are uncertain. In simian and probably in human malaria, recovery is known to require the presence of both T and B lymphocytes. It is probable that the T lymphocytes act partially through their helper effect on antibody production. Some authorities have suggested that they also play a direct role through lymphokine production by stimulating effector cells to release nonspecific factors capable of inhibiting intraerythrocytic multiplication. The B lymphocytes begin production of stage-and strain-specific antiplasmodial antibodies within the first 2 weeks of parasitemia. With the achievement of high levels of antibodies, the number of circulating parasites decreases. The infrequency with which malaria occurs in young infants has been attributed to the transplacental passage of such antibodies. It is uncertain whether they are directly lethal, act as opsonizing agents, or block merozoite invasion of RBCs. Antibody responses are also detectable against sporozoites and, because of this, much attention has been given to develop a vaccine against this parasite stage. Because sporozoites clear so quickly from the peripheral circulation, however, they may escape immune detection and all it would take is one to initiate hepatic schizogony resulting in blood stage infection. Antibodies against sporozoites have no effect on erythrocytic stages of infection.
Antibody-mediated immunity important
In simian malaria, the parasite can undergo antigenic variation and thereby escape the suppressive effect of the antibodies. This antigenic variation leads to cycles of recrudescent parasitemia, but ultimately to production of specific antibodies to the variants, and cure. In P falciparum malaria, chronic infection is maintained through the insertion of highly polymorphic variant antigens that are inserted into the infected erythrocyte membrane. With P falciparum, the disease typically does not exceed 1 year, but with P malariae the erythrocytic infection can be extremely persistent, lasting in one case up to 53 years. How erythrocytic parasites circulating in numbers too small to be detected on routine blood films escape immunologic destruction remains a puzzle. In a closely related simian malaria, splenectomy results in rapid cure, suggesting that suppressor T lymphocytes in the spleen may play a protective role. In infection with P vivax and P ovale, latent hepatic infection may result in the discharge of fresh merozoites into the bloodstream after the disappearance of erythrocytic forms. This phenomenon, known as relapse, is capable of maintaining infection for 3 to 5 years or longer.
Antigenic variation could play a role in persistence
In almost all cases, immunity to malaria is usually short lived and does not result in a sterile immunity. Many individuals, living in areas where transmission is sporadic, can be infected multiple times by the same species of parasite. A question often asked: If natural infection with malaria does not result in a lasting or sterile immunity, can a vaccine be developed that will?
MALARIA: CLINICAL ASPECTS
MANIFESTATIONS
The incubation period between the bite of the mosquito and the onset of disease is approximately 2 weeks. With P malariae and with strains of P vivax in temperate climates, however, this period is often more prolonged. Individuals who contract malaria while taking antimalarial suppressants may not experience illness for many months. In the United States, the interval between entry into the country and onset of disease exceeds 1 month in 25% of P falciparum infections and 6 months in a similar proportion of P vivax cases.
Incubation period prolonged by suppressant use
The clinical manifestations of malaria vary with the species of plasmodia but typically include chills, fever, splenomegaly, and anemia. The hallmark of disease is the malarial paroxysm. This manifestation begins with a cold stage, which persists for 20 to 60 minutes. During this time, the patient experiences continuous rigors and feels cold. With the consequent increase in body temperature, the rigors cease and vasodilatation commences, ushering in a hot stage. The temperature continues to rise for 3 to 8 hours, reaching a maximum of 40°C to 41.7°C before it begins to fall. The wet stage consists of a decrease in fever and profuse sweating. It leaves the patient exhausted but otherwise well until the onset of the next paroxysm.
Malarial paroxysm: Cold, hot, wet stages
Typical paroxysms first appear in the second or third week of fever, when parasite replication within erythrocytes becomes synchronized. In falciparum malaria, synchronization may never take place, and the fever may remain hectic and unpredictable. The first attack is often severe and may persist for weeks in the untreated patient. Eventually the paroxysms become less regular, less frequent, and less severe. Symptoms finally cease with the disappearance of the parasites from the blood.
Typical paroxysms after 2 to 3 weeks when parasite replication is synchronized
In falciparum malaria, capillary blockage can lead to several serious complications. When the central nervous system is involved (cerebral malaria), the patient may develop delirium, convulsions, paralysis, coma, and rapid death. Acute pulmonary insufficiency frequently accompanies cerebral malaria, killing about 80% of those involved. When splanchnic capillaries are involved, the patient may experience vomiting, abdominal pain, and diarrhea with or without bloody stools. Jaundice and acute renal failure are also common in severe illness. These pernicious syndromes generally appear when the intensity of parasitemia exceeds 100 000 organisms per cubic millimeter of blood. Most deaths occur within 3 days.
Cerebral falciparum malaria often lethal
DIAGNOSIS
Malarial parasites can be demonstrated in stained smears of the peripheral blood in virtually all symptomatic patients. Typically, capillary or venous blood is used to prepare both thin and thick smears, which are stained with Wright or Giemsa stain and examined for the presence of erythrocytic parasites. Thick smears, in which erythrocytes are lysed with water before staining, concentrate the parasites and allow detection of very mild parasitemia. Nonetheless, it may be necessary to obtain several specimens before parasites are seen. Artifacts are numerous in thick smears, and correct interpretation requires experience. The morphologic differences among the five species of plasmodia may allow their speciation on the stained smear by the skilled observer.
Thick and thin blood smears detect parasites
A number of attempts have been made to improve the standard thin and thick smear method. One such procedure involves acridine orange staining of centrifuged parasites in quantitative buffy coat (QBC) tubes. Although it is expensive, this requires a fluorescence microscope and permits less reliable parasite speciation; its rapidity and ease of use make it attractive to laboratories that are only occasionally called on to identify patients with malaria. Simple, specific card antigen detection procedures are now available. The most widely used test, ParaSight F, detects a protein (HRP2) excreted by P falciparum within minutes. The test can be performed under field conditions and has a sensitivity of more than 95%. A second rapid test, OptiMAL, detects parasite lactate dehydrogenase, and, unlike ParaSight F, can distinguish between P falciparum and P vivax. Numerous PCR assays have also been developed for the laboratory diagnosis of malaria.
Acridine orange stains and other rapid detection methods available
Serologic tests for malaria are offered at a few large reference laboratories but are used primarily for epidemiologic purposes. They are occasionally helpful in speciation and detection of otherwise occult infections. The recently completed sequencing of the malaria genome will lead to newer diagnostic methods.
TREATMENT
The indications for treatment rests on several factors. These include the severity of disease, the infecting species of Plasmodium, and the part of the world in which the infection was acquired. The immune status of the afflicted patient may also factor into this equation. The species and area of infection acquisition are likely to help determine if the parasite is resistant to any antimalarials or not. Falciparum malaria is potentially lethal in nonimmune individuals, such as new immigrants or travelers to a malarious area, and immunosup-pressed indigenous individuals, such as pregnant women. These individuals must be treated emergently.
The complete treatment of malaria requires the destruction of erythrocytic schizonts, hepatic schizonts, and erythrocytic gametocytes. The first terminates the clinical attack, the second prevents relapse, and the third renders the patient noninfectious to Anopheles and thus breaks the cycle of transmission. Unfortunately, no single drug accomplishes all three goals. The present strategy of chemotherapy is shown in Table 51–2.
Need to destroy all forms of the parasite
Termination of Acute Attack
Several agents can destroy asexual erythrocytic parasites. Chloroquine, a 4-aminoquino-line, has been the most commonly used. It acts by inhibiting the degradation of hemoglobin, thereby limiting the availability of amino acids necessary for growth. It has been suggested that the weak basic nature of chloroquine also acts to raise the pH of the food vacuoles of the parasite, inhibiting their acid proteases and effectiveness. When originally introduced, it was rapidly effective against all four species of plasmodia and, in the dosage used, free of serious side effects. However, chloroquine-resistant strains of P falciparum are now widespread in Africa and Southeast Asia; they are also found, though less frequently, in other areas of Asia and in Central America and South America. Chloroquine-resistant strains of P vivax have been reported from Papua New Guinea, India, and Pakistan, but overall remains poorly defined worldwide.
Other schizonticidal agents include quinine/quinidine, antifolate–sulfonamide combinations, mefloquine, halofantrine, and the artemisinins. Unfortunately, resistance to all of these agents is increasing, particularly in Southeast Asia. The artemisinins are also unique in their capacity to reduce transmission by preventing gametocyte development. Resistance to this latter first-line drug is increasing in areas of Southeast Asia.
Chloroquine inhibits hemoglobin degradation by parasite
Artemisinins prevent gametocyte development
Strains of P malariae, P ovale, and P vivax (except for some acquired in the South Pacific and South America) remain sensitive to chloroquine and may be treated with this agent. Plasmodium vivax infections acquired in New Guinea and Sumatra, however, should be assumed to be chloroquine-resistant and managed with mefloquine alone or in combination with other agents. Plasmodium falciparum has now become variably resistant to all drug groups, including the artemisinin compounds.
Resistance of chloroquine and other drugs now common with P falciparum
There is a growing consensus that the most effective way to slow the further development of drug-resistant strains of P falciparum is to use one of the artemisinins in combination with quinine/quinidine, antifolate–sulfonamide compounds, mefloquine, or halofantrine.
Combination therapy may be necessary
Radical Cure
In P vivax and P ovale infections, hepatic schizonts persist and must be destroyed to prevent reseeding of circulating erythrocytes with consequent relapse. Primaquine, an 8-amino-quinoline, is used for this purpose. Some P vivax infections acquired in Southeast Asia and New Guinea fail initial therapy owing to relative resistance to this 8-aminoquinoline. Retreatment with a larger dose of primaquine is usually successful. Unfortunately, prima-quine may induce hemolysis in patients with G6PD deficiency. Persons of Asian, African, and Mediterranean ancestry should thus be screened for this abnormality before treatment. Chloroquine destroys the gametocytes of P vivax, P ovale, and P malariae but not those of P falciparum. Primaquine and artemisinins, however, are effective for this latter species.
Primaquine used to destroy hepatic schizonts of P vivax and P ovale
PREVENTION
Personal Protection
In endemic areas, mosquito contact can be minimized with the use of house screens, insecticide bombs within rooms, and/or insecticide-impregnated mosquito netting around beds. Those who must be outside from dusk to dawn, the period of mosquito feeding, should apply insect repellent and wear clothing with long sleeves and pants. In addition, it is possible to suppress clinical manifestations of infection, if they occur, with a weekly dose of chloroquine. In areas where chloroquine-resistant strains are common, an alternative schizonticidal agent should be used. Mefloquine or doxycycline are usually preferred. The antifolate pyrimethamine plus a sulfonamide can be taken as well. However, use of this combination is occasionally accompanied by serious side effects, so it is recommended only when mefloquine- and doxycycline-resistant strains are present in the area, and then only for individuals residing in areas of intense transmission for prolonged periods of time. On leaving an endemic area, it is necessary to eradicate residual hepatic parasites with prima-quine before discontinuing suppressive therapy.
Mosquito protection with screens and repellents
Chemoprophylaxis choice must consider resistance in area
General
Malaria control measures have been directed toward reducing the infected human and mosquito populations to below the critical level necessary for sustained transmission of disease. The techniques used include those mentioned previously, treatment of febrile patients with effective antimalarial agents, chemical or physical disruption of mosquito breeding areas, and residual insecticide sprays. An active international cooperative program aimed at the eradication of malaria resulted in a dramatic decline in the incidence of the disease between 1956 and 1968. Eradication was not achieved, however, because mosquitoes became resistant to some of the chemical agents used, and today malaria still infects 200 to 300 million inhabitants of Africa, Latin America, and Asia. Tropical Africa alone accounts for 100 million of the afflicted and for most of the 1 million deaths that occur annually as a result of this disease. The long-term hope for progress in these areas now depends on the compliant use of existing and development of new technologies.
Reduce human reservoir and eradicate mosquitoes
Attempts at eradication have failed
Vaccines
Three advances in the last decade have produced the hope for the first time that an effective malaria vaccine might be within reach of medical science. The establishment of a continuous in vitro culture system provided the large quantities of parasite needed for antigenic analysis. Development of the hybridoma technique allowed the preparation of monoclonal antibodies with which antigens responsible for the induction of protective immunity could be identified. Finally, recombinant DNA procedures enabled scientists to clone and sequence the genes encoding such antigens, permitting the amino acid structure to be determined and peptide sequences suitable for vaccine development to be identified. In 2012, a phase III clinical trial consisting of a protein fragment from the outer surface of P falciparum, fused with a hepatitis B virus protein, and combined with an immune adjuvant reduced episodes of both clinical and severe malaria in children aged 5 to 17 months by approximately 50%. This vaccine targets the preerythrocytic stage of the disease. Studies are continuing, with development of new adjuvants that may be even more potent. Hopefully, this may lead to vaccine strategies that are sorely needed throughout the developing world.
Subunit vaccines fused with a hepatitis B protein have shown promise
Babesia
The genus Babesia is represented by species that are close relatives of malaria belonging to the order Piroplasmida. They are small parasites of the mammalian host RBCs and are transmitted by ticks. These parasites were the first shown to be transmitted by an arthropod intermediate host. The organism involved in this instance was B bigemina, the causative agent of redwater fever in cattle.
Babesia microti is the parasite of interest to human health. It was first reported from a patient on Nantucket Island, Massachusetts. Since then there have been hundreds of cases reported from New England and in the states of Wisconsin, Washington, and California. Hard ticks of the genus Ixodes are the principal vectors. These ticks are also capable of transmitting Lyme disease. Within the tick vector the disease can also be transmitted between stages of development (transstadial transmission) or across generations through the ova (transovarial transmission).
Unlike malaria, Babesia only infects the RBCs of its human host. Resulting symptoms can be flu-like with attendant symptoms of fevers, chills, sweats, etc; not too unlike malaria. Diagnosis is effected by finding the small piroplasms in blood smears. Because they resemble malaria it is often necessary to send smears to a reference laboratory or to have serologic or PCR testing performed.
Patients usually respond well to treatment with quinine and clindamycin. Because these may be poorly tolerated, atovaquone and azithromycin can also be used. Preventive measures include avoidance of areas known to be tick infected, using appropriate insecticides, wearing appropriate clothing and performing daily tick inspections if one ventures into wooded areas where ticks live.
TOXOPLASMA GONDII
PARASITOLOGY
Like the plasmodia, Toxoplasma gondii, the cause of toxoplasmosis, is an obligate intracellu-lar apicomplexan. It differs from Plasmodium in that both sexual and asexual reproductive cycles occur within the gastrointestinal tract of felines, the definitive host. The disease is transmitted to other host species by the ingestion of oocysts passed in the feces of infected felines, or through carnivorism from one infected host to another. The principal mode of transmission to humans is via ingestion of meat products containing tissue cysts (bradyzo-ites). Transplacental transmission may also occur.
Asexual and sexual cycles in felines
Spread to humans from felines via fecal-oral route and via ingestion of meat
MORPHOLOGY
Toxoplasma gondii was first demonstrated in 1908 in the gondi, an African rodent, by Nicolle and Manceaux. Its name, derived from the Greek toxo (arc), is based on the characteristic shape of the organism. All strains of this parasite appear to be closely related antigenically. The major morphologic forms of the parasite are the oocyst, trophozoite, and tissue cyst (Figure 51–6).
FIGURE 51–6. Toxoplasma gondii. A. Invasive trophozoite forms. B. Cyst in tissue. (Reproduced with permission from Nester EW: Microbiology: A Human Perspective, 6th edition. 2009.)
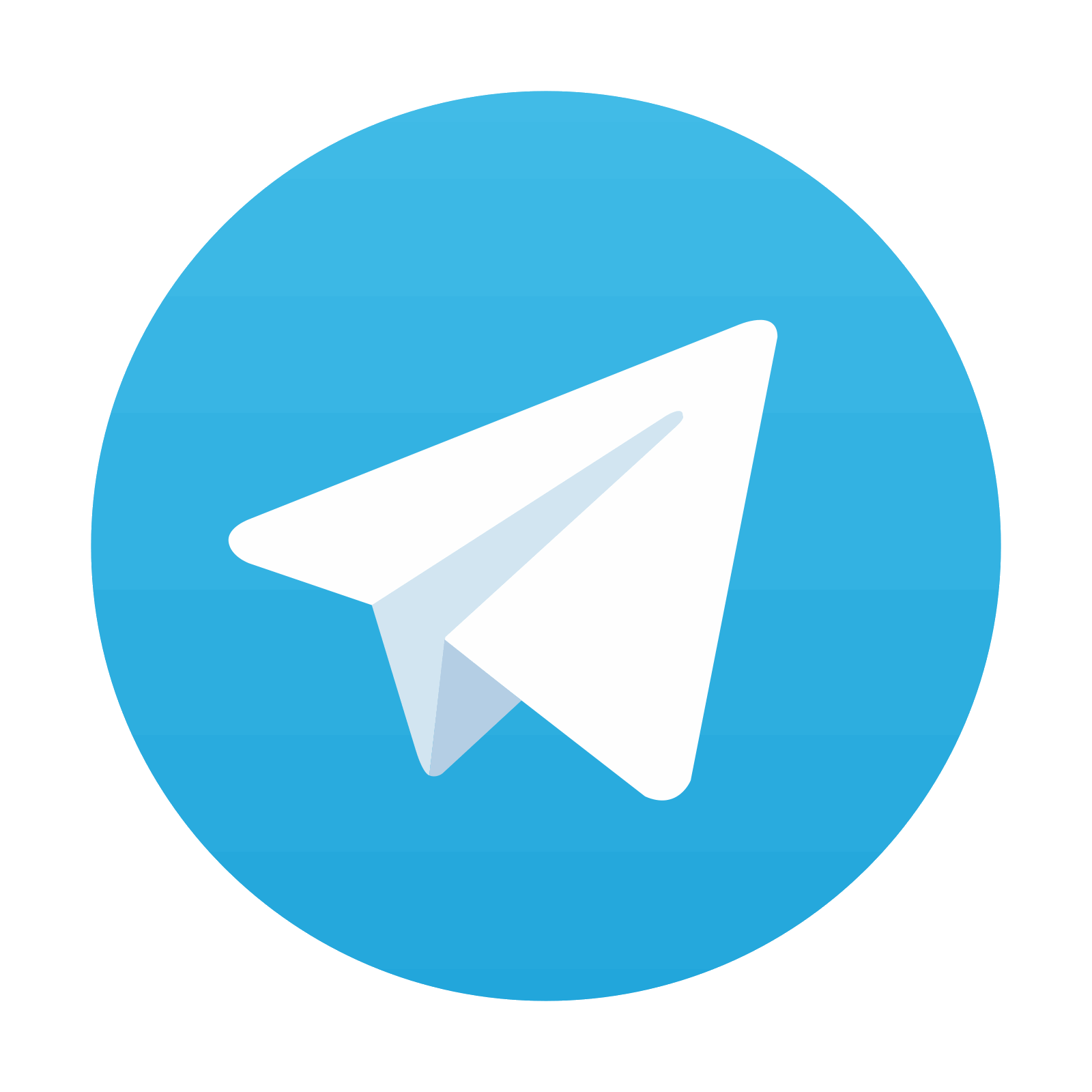
Stay updated, free articles. Join our Telegram channel
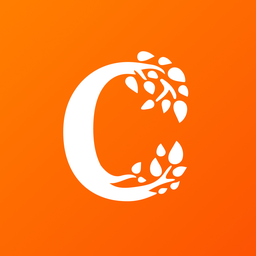
Full access? Get Clinical Tree
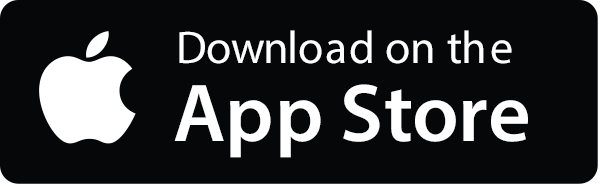
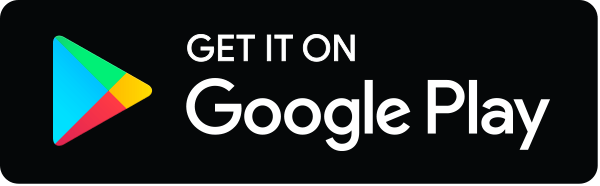