CHAPTER OUTLINE
Apolipoprotein A-IV and the Control of Feeding Behaviors
Hypothalamic apoA-IV and Satiety
Antioxidant and Anti-inflammatory Activities of HDL
Therapeutic Benefits of Elevating HDL
High-Yield Terms
Lipoprotein: complex of variable protein and lipid composition responsible for transport of fats throughout the body
Apolipoprotein: lipid-binding proteins of lipoprotein complexes
Apolipoprotein A-IV (apoA-IV): synthesized in intestines and hypothalamus, synthesis increased upon fat consumption, inhibits desire for food intake
Chylomicron: lipoprotein complex generated from dietary lipids within the enterocytes of the intestines
VLDL: lipoprotein complex generated from endogenous (or dietary) lipids within the liver, circulation in the vasculature leads to progressive loss of fatty acids converting these lipoproteins to IDL and finally LDL
HDL: high-density lipoprotein involved in the removal of cholesterol from peripheral tissue for return to the liver; the process is referred to as reverse cholesterol transport (RCT)
Space of Disse: the space between hepatic sinusoidal endothelium and hepatocytes, LDL and chylomicron remnants can be modified here prior to binding receptors on hepatocytes
Acyl-CoA-cholesterol acyltransferase (ACAT): key intracellular enzyme for esterifying cholesterol
Lecithin:cholesterol acyltransferase (LCAT) ratio: key HDL-associated enzyme involved in the esterification of cholesterol, removed from peripheral tissue, prior to exchange for triglyceride with VLDL and LDL
Cholesterol ester transfer protein (CETP): HDL-associated protein that transfers cholesterol esters from HDLs to VLDL and LDL in exchange of triglycerides from the VLDL/LDL to HDL
Paraoxonases (PON): HDL-associated enzymes that impart antioxidant activity of HDL toward LDL
Lipoprotein-associated phospholipase A2 (Lp-PLA2): is one of 2 forms of platelet-activating factor acetylhydrolase (PAF-AH), major HDL-associated hydrolase that is responsible for the hydrolysis of oxidized phospholipids
Lipoprotein(a) (Lp[a]): clinically significant apolipoprotein disulfide bonded to apoB-100 in LDL, easily oxidized and phagocytosed by macrophages-enhancing pro-inflammatory responses, inhibits the process of fibrin clot dissolution
Biochemically, a lipoprotein represents a complex assembly of both proteins and lipids. The major lipoproteins are those found in the plasma and are responsible for the mobilization of lipids, primarily fats, throughout the body. The lipid portion of lipoproteins consists of cholesterol, cholesterol esters, triglycerides, and/or phospholipids. Lipoproteins can be derived from dietary lipids via synthesis in enterocytes of the intestine or from endogenous lipids via synthesis in the liver. The generalization of lipoprotein types is defined by the amount of protein and lipid as depicted in Table 28-1.
The proteins associated with lipoproteins are referred to as apolipoproteins or often just apoproteins. Different apolipoproteins are found in functionally distinct lipoproteins types. There are 2 major types of apolipoproteins distinguished by the secondary structural motifs present in the proteins. The apolipoprotein A (apoA) proteins are composed primarily of alpha helices. These apoproteins form reversible complexes with the lipids of lipoproteins. The apolipoprotein B (apoB) proteins are composed primarily of β-sheet structures and form irreversible complexes with the lipids of lipoproteins. In addition to the apoA and apoB family of proteins, there are apoC, apoD, and apoE proteins. Several functionally significant apolipoproteins are described in Table 28-2.
Apolipoprotein A-IV and the Control of Feeding Behaviors
Apolipoprotein A-IV (apoA-IV) is synthesized exclusively in the small intestine and the hypothalamus. Intestinal synthesis of apoA-IV increases in response to ingestion and absorption of fat, and it is subsequently incorporated into chylomicrons and delivered to the circulation via the lymphatic system. Systemic apoA-IV has been shown to have effects in the CNS involving the sensation of satiety.
Intestinal apoA-IV
Following the consumption of fat, the absorption of the lipid content stimulates the synthesis and secretion of apoA-IV by the intestines. Chylomicrons serve as the inducing signal for apoA-IV transcription and secretion; however, the precise mechanism by which the transcriptional enhancement is affected is currently undetermined. A signal is released by the distal gut during active lipid absorption, which is capable of stimulating apoA-IV synthesis in the proximal gut and this signal is the ileal peptide protein tyrosine tyrosine (PYY).
Chronic consumption of a high-fat diet leads to significant reduction in apoA-IV mRNA levels and the response of hypothalamic apoA-IV gene expression to dietary lipids. Therefore, it is highly likely that dysregulation of hypothalamic apoA-IV could contribute to diet-induced obesity.
Although transcription of apoA-IV increases rapidly following fat ingestion, within 90 minutes the level of apoA-IV transcription is once again reduced. This reduction is a result of increased leptin secretion. Although numerous studies have demonstrated a negative correlation between leptin levels and apoA-IV expression, the mechanism by which this effect is exerted is not fully understood. There are leptin receptors in the gut and, therefore, leptin binding to these receptors could lead to direct effects on intestinal enterocytes.
Hypothalamic apoA-IV and Satiety
Expression of apoA-IV in the hypothalamus, a site intimately involved in regulating energy homeostasis, indicates that the effects exerted, on appetite, by apoA-IV are due to direct hypothalamic synthesis and secretion. This can be demonstrated by blocking apoA-IV actions by central injection of antibodies to the protein. Experiments of this type result in increased food consumption in experimental animals. Additional studies have shown that apoA-IV is involved in inhibiting food intake following the ingestion of fat. When apoA-IV containing chylomicrons are infused, there is marked suppression of food intake during the first 30 minutes following administration. If apoA-IV is removed from the chylomicrons prior to infusion, there is no observed effect on food intake. Alternatively, if apoA-IV itself is infused, suppression of food intake is the same as that seen with infusion of chylomicrons.
Chylomicrons
Chylomicrons are assembled in intestinal enterocytes as a means to transport dietary cholesterol and triglycerides to the rest of the body. Chylomicrons are, therefore, the molecules formed to mobilize dietary (exogenous) lipids (see Figure 28-1). The predominant lipids of chylomicrons are triglycerides (see Table 28-1). The apolipoproteins that predominate before chylomicrons enter the circulation include apoB-48, apoA-I, apoA-II, and apoA-IV, where apoB-48 is found exclusively in chylomicrons.
FIGURE 28-1: Structure of a chylomicron as a representative structure of a typical lipoprotein particle. Image demonstrates the outer layer of phospholipid and free cholesterol with primarily triglycerides and cholesteryl esters internally. Included in chylomicrons and other lipoprotein cores but not shown are triglycerides (TG). Each lipoprotein type, chylomicron, LDL, and HDL, contains apolipoproteins. Apolipoprotein B-48 (apoB-48) is specific for chylomicrons just as apoB-100 is specific for LDL. Reproduced with permission of themedicalbiochemistrypage, LLC.
Chylomicrons leave the intestine via the lymphatic system and enter the circulation at the left subclavian vein. In the bloodstream, chylomicrons acquire apoC-II and apoE from plasma HDL. In the capillaries of adipose tissue and muscle, the fatty acids of chylomicrons are removed from the triglycerides by the action of lipoprotein lipase (LPL), which is found on the surface of the endothelial cells of the capillaries (Figure 28-2). The apoC-II in the chylomicrons activates LPL in the presence of phospholipid. During the removal of fatty acids, a substantial portion of phospholipid apoA and apoC is transferred to HDL. The loss of apoC-II prevents LPL from further degrading the chylomicron remnants.
FIGURE 28-2: Metabolic fate of chylomicrons. (A, apolipoprotein A; B-48, apolipoprotein B-48; C, cholesterol and cholesteryl ester; E, apolipoprotein E; HDL, high-density lipoprotein; HL, hepatic lipase; LRP, LDL-receptor-related protein; PL, phospholipid; TG, triacylglycerol.) Only the predominant lipids are shown. Murray RK, Bender DA, Botham KM, Kennelly PJ, Rodwell VW, Weil PA. Harper’s Illustrated Biochemistry, 29th ed. New York, NY: McGraw-Hill; 2012.
Chylomicron remnants, containing primarily cholesterol esters, apoE and apoB-48, are then delivered to, and taken up by, the liver. The remnant particle must be of a sufficiently small size such that can pass through the fenestrated endothelial cells lining the hepatic sinusoids and enter into the space of Disse. Chylomicron remnants can then be taken up by hepatocytes via interaction with the LDL receptor, which requires apoE (Figure 28-3). In addition, in the space of Disse chylomicron remnants can accumulate additional apoE that is secreted free into the space. This latter process allows the remnant to be taken up via the chylomicron remnant receptor, which is a member of the LDL receptor-related protein (LRP) family. The recognition of chylomicron remnants by the hepatic remnant receptor also requires apoE. Chylomicron remnants can also remain sequestered in the space of Disse by binding of apoE to heparan sulfate proteoglycans and/or binding of apoB-48 to hepatic lipase. While sequestered, chylomicron remnants may be further metabolized, which increases apoE and lysophospholipid content allowing for transfer to LDL receptors or LRP for hepatic uptake.
FIGURE 28-3: Uptake of chylomicron remnants by the liver. The space between hepatic sinusoidal endothelium and hepatocytes is called the space of Disse. Chylomicron remnants containing primarily cholesterol esters apoE, and apoB-48 are rapidly taken up by the liver. The remnants that pass through the endothelial lining of the hepatic sinusoid in the space of Disse interact with specific receptors as well as heparin-sulfated proteoglycans (HSPG). Hepatocyte uptake of remnants is initiated by sequestration of the particles on HSPG followed by receptor-mediated endocytosis of the remnants. The receptor-mediated endocytic process may be mediated by LDL receptors (LDLR) and/or LDL receptor-related protein (LRP). The interaction of remnants with HSPG involves apoB-48 and the interaction with LDLR or LRP involves apoE. Reproduced with permission of themedicalbiochemistrypage, LLC.
VLDL, IDL, and LDL
Whereas chylomicrons represent the form of lipoprotein complex responsible for dietary lipid transport, very low density lipoproteins (VLDL) are the complexes involved in the transport of lipid (primarily triglyceride) from the liver to the extrahepatic tissues. In addition to triglycerides, VLDL contain some cholesterol and cholesterol esters, phospholipids, and the apoproteins, apoB-100, apoC-I, apoC-II, apoC-III, and apoE. Like nascent chylomicrons, newly released VLDL obtain apoC and apoE from circulating HDL. The fatty acid portion of VLDL is released into adipose tissue and muscle in the same way as for chylomicrons, through the action of LPL (Figure 28-4). The action of LPL, coupled with the transfer of apoC to HDL, converts VLDL to intermediate-density lipoproteins (IDL), also termed VLDL remnants. Further loss of triglycerides converts IDL to LDL. The predominant remaining proteins are apoB-100 and apoE. The interaction of LDL with HDL (see next section) is the major means for the return of extrahepatic cholesterol to the liver.
FIGURE 28-4: Metabolic fate of very low density lipoproteins (VLDL) and production of low-density lipoproteins (LDL). (A, apolipoprotein A; B-100, apolipoprotein B-100; C, cholesterol and cholesteryl ester; E, apolipoprotein E; HDL, high-density lipoprotein; IDL, intermediate-density lipoprotein; PL, phospholipid; TG, triacylglycerol.) Only the predominant lipids are shown. It is possible that some IDL is also metabolized via the low-density lipoprotein receptor–related protein (LRP). Murray RK, Bender DA, Botham KM, Kennelly PJ, Rodwell VW, Weil PA. Harper’s Illustrated Biochemistry, 29th ed. New York, NY: McGraw-Hill; 2012.
The exclusive apolipoprotein of LDL is apoB-100. LDLs are taken up by cells via LDL receptor-mediated endocytosis. LDL receptor recognition of LDL requires the presence of both apoB-100 and apoE. The LDL receptor is also called the apoB-100/apoE receptor. The uptake of LDL occurs predominantly in liver (75%), adrenals, and adipose tissue. The interaction of LDL with LDL receptors requires the presence of apoB-100. The endocytosed membrane vesicles (endosomes) fuse with lysosomes, in which the apoproteins are degraded and the cholesterol esters are hydrolyzed to yield free cholesterol. The cholesterol is then incorporated into the plasma membranes as necessary. Excess intracellular cholesterol is re-esterified by acyl-CoA-cholesterol acyltransferase (ACAT) for intracellular storage.
High-Density Lipoproteins
HDLs represent a heterogeneous population of lipoproteins in that they exist as functionally distinct particles possessing different sizes, protein content, and lipid composition. HDLs are synthesized de novo in the liver and small intestine, as primarily protein-rich disc-shaped particles. These newly formed HDLs are nearly devoid of any cholesterol and cholesterol esters. The primary apoproteins of HDLs are apoA-I, apoC-I, apoC-II, and apoE. In fact, a major function of HDL is to act as circulating stores of apoC-I, apoC-II, and apoE. ApoA-I is the most abundant protein in HDL constituting over 70% of the total protein mass.
In addition to RCT, HDLs exert anti-inflammatory, antioxidant, and vasodilatory effects that together represent additional atheroprotective functions of HDL. HDLs have also been shown to exert antiapoptotic, antithrombotic, and anti-infectious properties. In addition to apoproteins, HDLs carry numerous enzymes that participate in the antioxidant activities of the complex. These enzymes include glutathione peroxidase 1 (GPx-1), paraoxonase 1 (PON1), and platelet-activating factor acetylhydrolase (PAF-AH, also called lipoprotein-associated phospholipase A2, Lp-PLA2). With respect to the various atheroprotective functions of HDL, it is the small dense particles (referred to as HDL3) that are the most beneficial.
The primary mechanism by which HDLs acquire peripheral tissue cholesterol is via an interaction with monocyte-derived macrophages in the subendothelial spaces of the tissues (Figure 28-5). Macrophages bind nascent HDL, that contains primarily apoA-I, through interaction with the ATP-binding cassette transport protein A1 (ABCA1). The transfer of cholesterol from macrophages via the action of ABCA1 results in the formation of nascent discoidal lipoprotein particles termed pre-β HDL. The free cholesterol transferred in this way is esterified by HDL-associated lecithin:cholesterol-acyltransferase (LCAT) ratio. LCAT is synthesized in the liver and so named because it transfers a fatty acid from the C–2 position of lecithin to the C–3–OH of cholesterol, generating a cholesterol ester and lysolecithin. The activity of LCAT requires interaction with apoA-I. The cholesterol esters formed via LCAT activity are internalized into the hydrophobic core of the pre-β HDL particle. As pre-β HDL particles enlarge with progressive uptake of cholesterol, they become larger and spherical generating the HDL3 and then HDL2 particles. HDLs also extract cholesterol from cell surface membranes, which involves the action of the ATP-binding cassette protein G1 (ABCG1). Approximately 20% of HDL uptake of peripheral tissue cholesterol occurs via the ABCG1-mediated pathway. The importance of ABCA1 in RCT is evident in individuals harboring defects in ABCA1 gene. These individuals suffer from a disorder called Tangier disease, which is characterized by 2 clinical hallmarks; enlarged lipid-laden tonsils and low-serum HDL (see Clinical Box 28-1). Cholesterol-rich HDLs return to the liver, where they bind to a receptor that is a member of the scavenger receptor family, specifically the scavenger receptor B1 (SR-B1). HDL binding to SR-B1 does not result in internalization as in the case of LDL receptor-mediated endocytosis. The cholesterol esters of HDLs are taken up by the hepatocytes through caveolae while the HDLs and SR-B1 remain on the plasma membrane.
FIGURE 28-5: Details of the interactions between HDL and LDL within the vasculature. As indicated in the text, HDL begins as protein-rich discoidal structures, composed primarily of apoA-I, produced by the liver and intestines. Within the vasculature, apoA-I interacts with the ATP-binding cassette transporter, ABCA1 (such as is diagrammed for interaction with macrophages) and extracts cholesterol from cells. Through the action of LCAT, the apoA-I–associated cholesterol is esterified forming cholesterol esters. This process results in the generation of HDL3 particles. As the HDL3 particles continue through the circulation, they pick up more cholesterol and through the action of LCAT, generate more cholesterol esters. As HDL migrates through the vasculature, there is an interaction between them and IDL and LDL. This interaction occurs through the action of CETP, which exchanges the cholesterol esters in the HDL for triglycerides from LDL. This interaction results in the conversion of HDL3 particles to HDL2. The differences between these 2 types of HDL particle are detailed in Table 28-1. HDL can also remove cholesterol from cells via interaction with the ATP-binding cassette transporter ABCG1. Approximately 20% of HDL uptake of cellular cholesterol occurs via ABCG1. HDL is then removed from the circulation by the liver through binding of the HDL to hepatic scavenger receptor SR-B1. Cholesterol ester–rich IDL and LDL can return to the liver and be taken up through interaction with the LDL receptor (LDLR). Within the vasculature, the generation of ROS results in oxidation of lipid components of LDL, generating oxidized LDL (oxLDL) that is taken up by the macrophages via the scavenger receptor, FAT/CD36. Reproduced with permission of themedicalbiochemistrypage, LLC.
CLINICAL BOX 28-1: TANGIER DISEASE
Tangier disease (TD) is an autosomal recessive disorder resulting from defects in the ATP-binding cassette (ABC) transporter family gene encoding ABCA1. The disease is so named because it was originally identified in 2 individuals living on Tangier Island, Virginia. Tangier disease is a rare disease with only around 50 cases identified worldwide. Although a rare disease, the identification of the gene defective in the disease and an analysis of the function of the encoded transporter protein have provided insight into overall cellular cholesterol homeostasis. Expression of the ABCA1 gene is highest in placenta, liver, lung, and adrenal glands. Numerous pathways of transcriptional regulation of ABCA1 have been identified including cAMP, nuclear receptors (such as LXRs, RXRs, and PPARs), hormones, and cytokines. The involvement of cytokines in the regulation of ABCA1 expression demonstrates the crosstalk between cellular cholesterol management and inflammatory responses. Pro-inflammatory cytokines such as tumor necrosis factor-α (TNF-α) and interleukin-1β (IL-1β) interfere with the transcriptional enhancement of ABCA1 exerted by LXRs. The predominant site of ABCA1 protein localization is the plasma membrane. In nonhepatic tissue, the role of ABCA1 is to promote the efflux of cholesterol principally to the HDL class of lipoprotein particles, which in turn transport the cholesterol back to the liver for catabolism. The clinical hallmarks of Tangier disease are a lack of HDL and apoA-I. The clinical manifestations of Tangier disease are related to an accumulation of cholesterol esters in reticuloendothelial tissues (eg, tonsils, lymph nodes, spleen, thymus, and bone marrow). The first symptom seen in almost all Tangier disease patients is enlarged tonsils that appear orange in color. Additional typical clinical findings include splenomegaly, a variable incidence of cardiovascular disease, and peripheral neuropathy.
High-Yield Concept
The consumption of alcohol is associated with either a protective or a negative effect on the level of circulating LDL. Low-level alcohol consumption, particularly red wines, which contain the antioxidant resveratrol, appear to be beneficial with respect to cardiovascular health. Resveratrol consumption is associated with a reduced risk of cardiovascular, cerebrovascular, and peripheral vascular disease. One major effect of resveratrol in the blood is the prevention of oxidation of LDL, forming oxidized LDL (oxLDL). OxLDL contribute significantly to the development of atherosclerosis. Conversely excess alcohol consumption is associated with the development of fatty liver, which in turn impairs the ability of the liver to take up LDL via the LDL receptor resulting in increased LDL in the circulation.
One of the major functions of HDL is to acquire cholesterol from peripheral tissues and transport this cholesterol back to the liver, where it can ultimately be excreted following conversion to bile acids. This function is referred to as reverse cholesterol transport (RCT). The role of HDL in RCT represents the major atheroprotective (prevention of the development of atherosclerotic lesions in the vasculature) function of this class of lipoprotein.
Reverse cholesterol transport also involves the transfer of cholesterol esters from HDL to VLDL and LDL. This transfer requires the activity of the plasma glycoprotein cholesterol ester transfer protein (CETP). The transfer of cholesterol esters from HDL to VLDL involves a reciprocal exchange of triglycerides from the VLDL to the HDL. This action allows excess cellular cholesterol to be returned to the liver through the LDL receptor. Additionally, when HDL particles become enriched with triglycerides they are better substrates for the action of hepatic lipase (see Figure 28-5).
In the absence of systemic inflammation, many of the enzymes and apolipoproteins associated with HDL play important roles in reducing the amount of oxidized lipid to which peripheral tissues are exposed. Some of these important proteins are apoA-I, PON1, GPx, and PAF-AH. However, when an individual has an ongoing systemic inflammatory state, these antioxidant proteins can be dissociated from the HDL or become inactivated resulting in the increased generation of oxidized and peroxidized lipids, which are proatherogenic.
Antioxidant and Anti-inflammatory Activities of HDL
In addition to their role in RCT, HDLs exert important anti-inflammatory and antioxidant properties within the vasculature. Various apolipoproteins associated with HDLs have been shown to be critical for these beneficial effects of HDL.
Apolipoprotein A-I: In addition to reverse cholesterol transport, apoA-I can remove oxidized phospholipids from oxidized LDL (oxLDL) and from cells. Specific methionine residues of apoA-I have been shown to directly reduce cholesterol ester hydroperoxides and phosphatidylcholine hydroperoxides.
Apolipoprotein A-II: ApoA-II–enriched HDLs support highly effective RCT from macrophages and also protect VLDL from oxidation more efficiently than apoA-II–deficient HDL. Recent clinical studies in human patients show that the higher the plasma apoA-II concentration, the lower is the risk of developing coronary heart disease (CHD).
Apolipoprotein A-IV: ApoA-IV has multiple activities related to lipid and lipoprotein metabolism as well as the control of feeding behaviors. ApoA-IV participates in RCT by promoting cholesterol efflux as well as by activation of LCAT. ApoA-IV has also been shown to have antioxidant, anti-inflammatory, and antiatherosclerotic actions.
Paraoxonases 1 and 3: Paraoxonases are a family of enzymes that hydrolyze organophosphates. Paraoxonase 1 (PON1) is synthesized in the liver and is carried in the serum by HDL. PON1 possesses antioxidant properties, in particular it prevents the oxidation of LDL. PON1 has been shown to enhance cholesterol efflux from macrophages by promoting HDL binding mediated by ABCA1, which in turn results in a reduction of pro-inflammatory signaling by these cells. In human clinical studies, a higher level of PON1 activity is associated with a lower incidence of major cardiovascular events. Other pathological conditions in humans that are associated with oxidative stress, such as rheumatoid arthritis and Alzheimer disease, are frequently associated with reduced activity of PON1. PON3, which is another HDL-associated paraoxonase, has also been shown to prevent the oxidation of LDL.
Platelet-activating factor acetylhydrolase (PAF-AH): There are 2 major forms of PAF-AH, cytosolic and plasma lipoprotein associated. The plasma form of PAF-AH (lipoprotein-associated PLA2, Lp-PLA2) circulates bound to HDL. Lp-PLA2 is a major HDL-associated hydrolase responsible for the hydrolysis of oxidized phospholipids. In humans, Lp-PLA2 deficiency is associated with increases in cardiovascular disease, while conversely circulating levels of Lp-PLA2 serve as an independent marker of the risk for developing CHD.
Glutathione peroxidase 1: Glutathione peroxidase 1 (GPx-1) has been shown to reduce lipid hydroperoxides to corresponding hydroxides, effectively detoxifying these types of abnormally modified lipids. Numerous human clinical studies indicated that GPx-1 provides a protective role against the development of atherosclerosis.
Sphingosine-1-phosphate (S1P): S1P is a bioactive lysophospholipid involved in a number of physiologically important pathways (see Chapter 21). HDLs are the most prominent carriers of S1P. Indeed, many of the biological effects of HDLs are mediated, in part, via S1P binding to its cell surface receptors. Effects of HDL on endothelial cells, such as migration, proliferation, and angiogenesis, are mediated, in part, by S1P associated with HDL. HDL-associated S1P inhibits pro-inflammatory responses, such as the generation of reactive oxygen species, activation of NAD(P)H oxidase, and the production of monocyte chemoattractant protein-1. While the HDL-associated forms of S1P exhibit these anti-inflammatory effects, free plasma S1P can activate inflammatory events dependent upon the receptor subtype to which it binds.
Therapeutic Benefits of Elevating HDL
Numerous epidemiological and clinical studies have demonstrated a direct correlation between the circulating levels of HDL cholesterol (most often abbreviated HDL-c) and a reduction in the potential for atherosclerosis and CHD. Individuals with levels of HDL above 50 mg/dL are several time less likely to experience CHD than individuals with levels below 40 mg/dL. In addition, clinical studies involving increases in circulating HDL levels correlate with a reduced incidence of CHD. Thus, there is precedence for therapies aimed at raising HDL levels in the treatment and prevention of atherosclerosis and CHD.
Cholesterol ester transfer protein (CETP) plays a critical role in HDL metabolism by facilitating the exchange of cholesterol esters from HDL for triglycerides in apoB–containing lipoproteins, such as LDL and VLDL (see Figure 28-5). The activity of CETP directly lowers the cholesterol levels of HDL and enhances HDL catabolism by providing HDL with the triglyceride substrate of hepatic lipase. Thus, CETP plays a critical role in the regulation of circulating levels of HDL, LDL, and apoA-I. The potential for the therapeutic use of CETP inhibitors in humans was first suggested when it was discovered in 1985 that a small Japanese population had an inborn error in the CETP gene leading to hyperalphalipoproteinemia and very high HDL levels. CETP inhibitors currently being tested in clinical trials are anacetrapib and dalcetrapib.
Lipoprotein Receptors
LDL Receptors
LDL returns cholesterol to the liver via the interaction of LDL with the LDL receptor (also called the apoB-100/apoE receptor). The sole apoprotein present in LDL is apoB-100, which is required for interaction with the LDL receptor. The LDL receptor is a polypeptide of 839 amino acids that spans the plasma membrane. An extracellular domain is responsible for apoB-100/apoE binding. The intracellular domain is responsible for the clustering of LDL receptors into regions of the plasma membrane termed coated pits. Once LDL binds the receptor, the complexes are rapidly internalized (endocytosed). ATP-dependent proton pumps lower the pH in the endosomes, which results in dissociation of LDL from the receptor. The LDL receptors are then recycled to the plasma membrane and the LDL-containing endosomes fuse with lysosomes. Acid hydrolases of the lysosomes degrade the apoproteins and release free fatty acids and cholesterol. The free cholesterol is either incorporated into plasma membranes or esterified (by ACAT) and stored within the cell.
LDL Receptor-Related Proteins
The LDL receptor-related protein (LRP) family represents a group of structurally-related transmembrane proteins involved in a diverse range of biological activities including lipid metabolism, nutrient transport, protection against atherosclerosis, as well as numerous developmental processes. The LDL receptor (LDLR) represents the founding member of this family of proteins. The LRP include LRP1, LRP1b, LRP2 (also called megalin), LRP4 (also called MEGF7 for multiple epidermal growth factor-like domains protein 7), LRP5/6, LRP8 (also called apolipoprotein E receptor 2), the VLDL receptor (VLDLR), and LR11/SorLA1 (LDL receptor relative with 11 ligand-binding repeats/sorting protein-related receptor containing LDLR class A repeats). LRP1 (also known as CD91 or α2-macroglobulin receptor) is expressed in numerous tissues and is known to be involved in diverse activities that include lipoprotein transport, modulation of platelet-derived growth factor receptor-β (PDGFRβ) signaling, regulation of cell-surface protease activity, and the control of cellular entry of bacteria and viruses. LRP1 has been shown to bind more than 40 different ligands that include lipoproteins, extracellular matrix proteins, cytokines and growth factors, protease and protease inhibitor complexes, and viruses. This diverse array of ligands clearly demonstrates that LRP1 is involved in numerous biological and physiological processes.
Scavenger Receptors
The founding member of the scavenger receptor family was identified in studies that were attempting to determine the mechanism by which LDL accumulated in macrophages in atherosclerotic plaques. Macrophages ingest a variety of negatively charged macromolecules that includes modified LDL, such as oxidized LDL (oxLDL). Subsequent research determined that the scavenger receptor family consists of several families that are identified as class A receptors, class B receptors, mucin-like receptors, and endothelial receptors. After binding ligand, the scavenger receptors can either be internalized, similar to the process of internalization of LDL receptors, or they can remain on the cell surface and transfer lipid into the cell through caveolae. The class A receptors include the type 1 and 2 macrophage scavenger receptors. The class B receptors include CD36 and scavenger receptor class B type 1 (SR-B1). The CD36 receptor is also known as fatty acid translocase (FAT) and it is one of the receptors responsible for the cellular uptake of fatty acids (see Chapter 23). CD36 and SR-B1 are closely related multi-ligand receptors and are recognized mostly for their roles in lipid and lipoprotein metabolism. The SR-B1 protein has been shown to be the endogenous receptor for HDL in the liver.
Abnormal Lipoproteins and the Lipoproteinemias
Lipoprotein (a) (Lp[a]) is composed of a common LDL nucleus linked to a molecule of apolipoprotein(a) (apo[a]) by disulfide bonds to apoB-100. When attached to apoB-100, the apo(a) protein surrounds the LDL molecule. Numerous epidemiological studies have demonstrated that elevated plasma levels of Lp(a) are a significant risk factor for the development of atherosclerotic disease (see Chapter 48). Apo(a) contains numerous secondary structures called Kringle domains and the protein exhibits a variability in size in different individuals due to a polymorphism caused by a variable number of the Kringle domains. The Kringle domains of apo(a) exhibit 75%-85% similarity to the Kringle domains of plasminogen, which is involved in the process of hemostasis (see Chapter 51).
When in the circulation, Lp(a) particles can be affected by oxidative modification similar to that of the other plasma lipoprotein particles. Lp(a) and oxidized Lp(a) (oxLp[a]) particles interact with macrophages via scavenger receptor uptake leading to cholesterol accumulation and foam cell formation. Indeed, oxLp(a) are phagocytosed more rapidly than other lipoprotein particles and, therefore, accumulate in the subendothelial space at high levels. This process can lead to progression of atherogenesis, thus accounting for the direct correlation between the plasma level of Lp(a) and coronary artery disease. In addition to oxidation of Lp(a) leading to increased foam cell production, glycation of the particle also may contribute to atherogenesis. In fact, there is a strong correlation in the level of glycated Lp(a) and the severity of hyperglycemia observed in poorly controlled type 2 diabetes.
Lp(a) has been shown to competitively inhibit the binding of plasminogen to its receptor on endothelial cells as well as to its binding sites on fibrinogen and fibrin. The normal function of plasminogen is to degrade the fibrin mesh in blood clots. This interference of plasminogen binding leads to reduced surface-dependent activation of plasminogen to plasmin (Figure 28-6). Therefore, high plasma concentrations of Lp(a) may represent a source of antifibrinolytic activity. In addition to the role of Lp(a) in inhibiting plasminogen binding, Lp(a) has been shown to inhibit the release of tissue plasminogen activator (t-PA) from endothelial cells. With reduced release of t-PA, there is decreased conversion of plasminogen to plasmin. This, along with the Lp(a)-mediated interference in plasminogen binding to fibrin clots results in a significant negative effect on the ability to dissolve blood clots. Lp(a) also stimulates the production of plasminogen activator inhibitor-1 (PAI-1), leading to a reduced ability of tissue t-PA to activate the process of clot dissolution.
FIGURE 28-6: Mechanism of Lp(a) interference with normal fibrinolysis. The apo(a) protein disulfide bonded to apoB-100 in LDL contains Kringle domains that interfere with plasminogen binding to fibrin clots and also interfere with the ability of tPA to hydrolyze plasminogen to active plasmin, thereby, interfering with the normal course of fibrin clot dissolution. Reproduced with permission of themedicalbiochemistrypage, LLC.
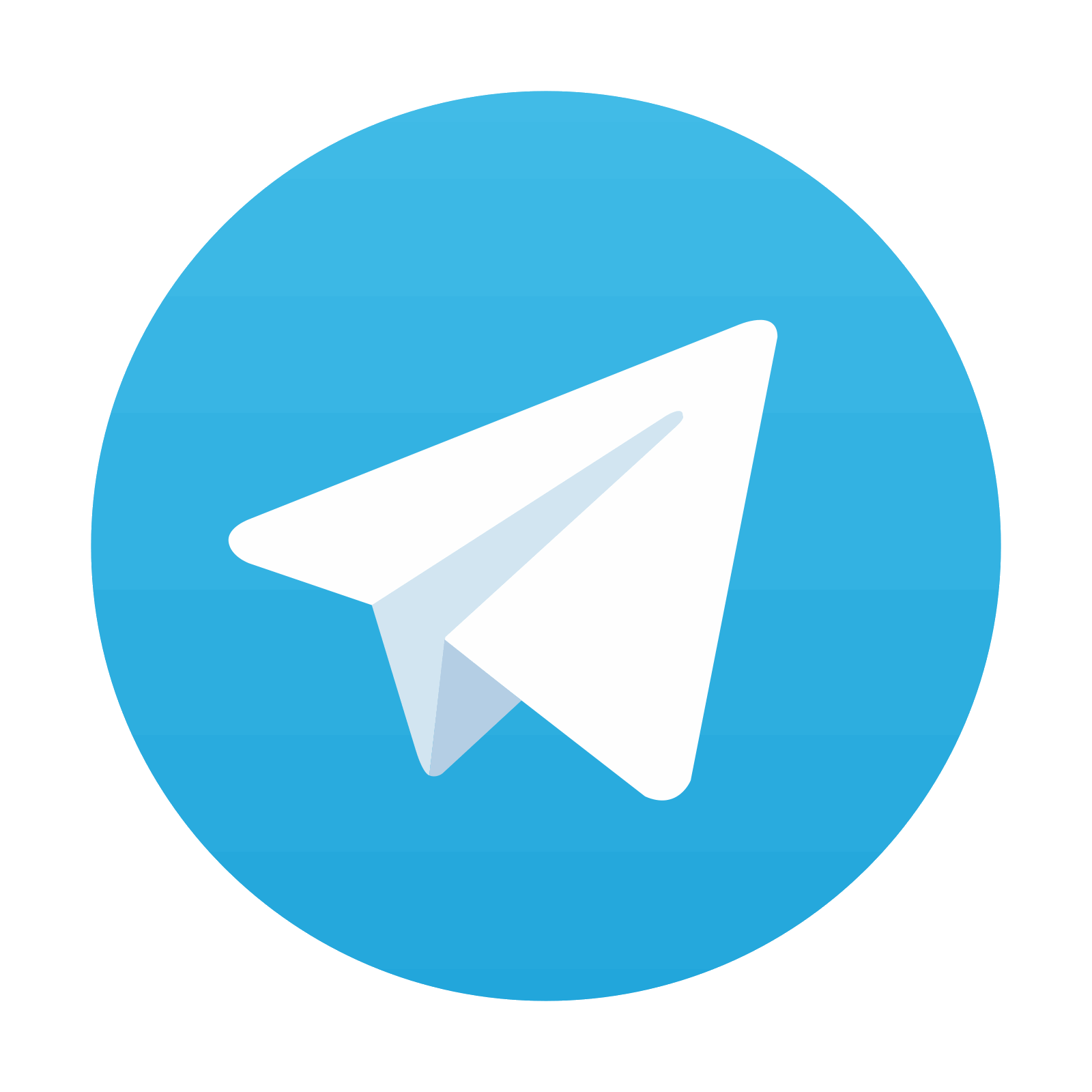
Stay updated, free articles. Join our Telegram channel
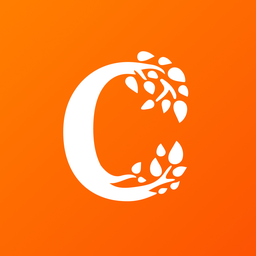
Full access? Get Clinical Tree
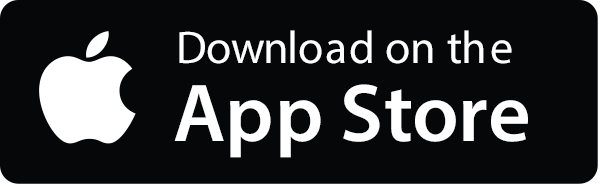
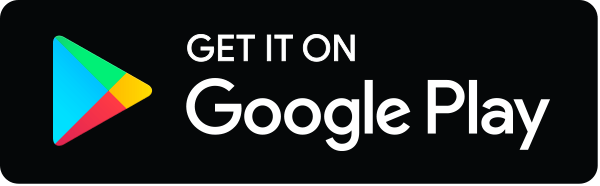