Zinc1
Janet C. King
Robert J. Cousins
1Abbreviations: AD, Alzheimer disease; AMD, age-related macular degeneration; EAR, estimated average requirement; FAO, Food and Agriculture Organization; GI, gastrointestinal; HIV, human immunodeficiency virus; IFN, interferon; Kd, dissociation constant; LOAEL, lowest observed adverse effect level; MT, metallothionein; NO, nitric oxide; RDA, recommended dietary allowance; UL, tolerable upper intake level; WHO, World Health Organization; ZnT, zinc transporter.
HISTORICAL BACKGROUND
Zinc (Zn) essentiality in plants was established in 1869, in experimental animals in 1934, and in humans in 1961. The biochemical basis for essentiality has not been established, but it rests within the catalytic, structural, and regulatory roles of this micronutrient. Detailed reviews are available (1, 2, 3, 4). A syndrome of anemia, hypogonadism, and dwarfism was reported in a 21-year-old Iranian man in 1961 who was subsisting on a diet of unrefined flat bread, potatoes, and milk. Shortly thereafter, a similar syndrome was observed in adolescent boys in Egypt who were also subsisting on a diet of flat bread and a few vegetables. Administration of a hospital diet improved growth and corrected the hypogonadism. Subsequent studies showed that the syndrome was primarily the result of a lack of zinc in the diet. Since the discovery of human zinc deficiency, interest in the biochemical and clinical aspects of zinc nutrition has increased exponentially.
CHEMISTRY
Zinc2+ (Zn2+) is a stronger Lewis acid (electron acceptor) than iron (Fe3+), but it is weaker than copper (Cu2+). This property favors strong binding to thiolate and amine electron donors (5). Zinc exhibits fast ligand exchange, which is believed to be important for some biochemical functions. Zinc does not exhibit redox chemistry directly, but the release of Zn2+ from a Zn-thiolate cluster by an oxidant produces disulfide bonds. Zn-sulfur (S) thiolate bonds, therefore, are sensitive to cellular redox (6).
Analytical procedures focus on atomic absorption spectrophotometry and inductively coupled plasma emission. Both have working ranges suitable for biologic samples and have generated much of the literature in this chapter. Zinc reference standards are available from the National Institute of Standards and Technology (NIST). Of the zinc radioisotopes, only 65Zn (half-life, 245 days) has been widely used in research. Stable isotopes of zinc and corresponding natural abundances are as follows: 64Zn, 49%; 66Zn, 29%; 67Zn, 4%; 68Zn, 19%; and 70Zn, 1%. These isotopes have been effectively used in experiments with humans. Highly specific fluorescent probes for zinc, such as FluoZin-3, are coming into wide use (7). Applications include studies of zinc transport by cells and organelles and measures of labile Zn2+ concentrations (a putative free Zn2+ pool) within cells (8).
BIOCHEMICAL AND PHYSIOLOGIC FUNCTIONS
Zinc-dependent biochemical mechanisms that determine physiologic functions have received extensive study, but clear relationships with phenotype have not been defined. Zinc has a ubiquitous subcellular distribution, which hampers this situation. Consequently, zinc contrasts with iron, which exists in defined cellular components and has defined physiologic roles. Three general functional classes—catalytic, structural, and regulatory—define zinc’s role in biology (9).
Catalytic Functions
Zinc serves a catalytic role in enzymes from all six enzyme classes (10). More than 300 zinc metalloenzymes have been identified. When the same enzyme identified in different species is counted only once, however, the number is much lower. How zinc is donated to apometalloenzymes has not been established. Zinc binding is a posttranslational protein modification, which likely requires a metal donor molecule or pH appropriate for zinc solubility, perhaps coordinated by events in the endoplasmic reticulum or a vesicular compartment. This process may require zinc transporter activity. An example is that a ZnT5/ZnT7 complex provides Zn2+ to activate tissue-nonspecific alkaline phosphatase (10).
An enzyme is generally considered a zinc metalloenzyme if the removal of zinc causes loss of activity without irreversibly altering the protein, and selective reconstitution with zinc restores the activity. Examples are the nucleotide polymerases (RNA polymerases I, II, and III), alkaline phosphatases, and the carbonic anhydrases. Unequivocal evidence of a direct link between signs of zinc deficiency or toxicity and a specific metalloenzyme has not been shown in complex organisms, and it is most likely an overt physiologic defect would occur only if the zinc-requiring enzyme was rate limiting in a critical biochemical pathway. Older literature has examples of relationships among zinc, enzyme, and disease (e.g., alcohol dehydrogenase and liver disease and RNA polymerases and growth retardation). Such enzyme changes are no longer considered as representing a critical function for zinc. Reports documenting zinc-responsive control of enzymes for intermediary metabolism, perhaps operating through effects on intracellular zinc concentrations, have been published (11, 12). Demonstrated physiologic control of some zinc transporters offers a new appreciation of the way in which coordinated Zn2+ fluxes in cells could influence enzyme activity (reviewed in 13).
Structural Functions
The structural function for zinc had its origin in 1985 with identification of a transcription factor having coordinating zinc-binding motifs (14). These motifs (“zinc fingers”) use cysteine and histidine to form a tetrahedral Zn2+ coordination complex. These have the general structure -C-X2-C-Xn-C-X2-C-, where C designates cysteine or histidine and X designates other amino acids. Zinc fingers have two to four cysteines and up to two histidines. Removal of zinc from zinc finger proteins alters folding and results in loss of function and probably degradation. Classic examples of zinc finger transcription factors are the retinoic acid and calcitriol nuclear receptors. The human transcriptome has 2500 zinc finger protein genes (15). This represents approximately 8% of the genome, a finding suggesting that a significant portion of the zinc requirement is allocated to maintain occupancy of zinc finger proteins. The mouse transcriptome has a comparable number of zinc finger genes (16). Binding affinity (apparent stability constants) of the fingers varies widely (dissociation constant [Kd] = 108 – 1011 M-1). By comparison, metallothionein (MT) binds zinc strongly (to a maximum Kd = 1012 M-1) (17). Both nitrosative stress and oxidative stress can disrupt zinc finger motifs and can cause loss of function, at least for oxidative stress (18). Because zinc fingers exhibit a spectrum of binding affinities (19), some sites may be particularly facile and potentially influenced by dietary zinc through zinc transporter activity.
Zinc finger proteins have a broad cell distribution and also bind RNA molecules and facilitate protein-protein interactions. These functions broaden their biologic role to include transcriptional and translational control, modulation of those processes, and signal transduction. Interest in zinc finger motifs is considerable because of their potential as targets for therapeutic interventions, including gene therapy.
Zn/S clusters, such as those in MT, may act as low redox potential units (17). These zinc thiol clusters, when oxidized by cellular oxidants (including oxidative and nitrosative stressors), results in zinc release. The glutathione/glutathione disulfide (GSH/GSSG) redox couple and some selenium compounds influence zinc release, which potentially integrates MT into cellular redox mechanisms. Nitric oxide (NO) may also mobilize zinc from this protein’s thiolate clusters (20, 21). This mobilization may be limited to the protein’s beta domain (3 Zn cluster), whereas the alpha domain (4 Zn cluster) is viewed as having a detoxifying role. Increased oxidative and nitrosative stress that accompanies zinc deficiency (22, 23) may be explained in part by induction of NO synthase (24, 25).
A hybrid function between structure and regulatory is that movement of large quantities of zinc are associated with insulin secretion by pancreatic β cells, zinc metallodigestive enzyme secretion by pancreatic acinar cells, and acid secretion by parietal cells of the stomach. In the first two instances, Zn2+ has a stabilizing role during the secretory process, whereas Zn2+ may replace hydrogen ion (H+) during gastric acid release (26, 27). The transporters
ZnT8, ZnT2, and ZIP11 are the likely major players for these functions.
ZnT8, ZnT2, and ZIP11 are the likely major players for these functions.
Regulatory Functions
Regulation of gene expression is a biochemical role for zinc. Originally identified as an active component of the metalloregulatory mechanism for MT gene regulation, the metalresponse element (MRE)-binding transcription factor 1 (MTF1) now is believed to provide zinc responsiveness to many genes (28, 29), including acting as a master regulatory transcription factors (30) for miRNA genes involved in gene repression. Null mutation of the MTF1 gene produces embryonic lethality in mice, a finding indicating importance in animals. On zinc occupancy, MTF1 translocates to the nucleus, where it participates in chromatin binding through MREs of the gene promoter. Polymorphisms in the zinc finger domain of the human MTF1 gene (31) suggest the possibility of genetic variation in the response of MTFl-regulated genes to dietary zinc intakes. A homologous transcription factor MTF2, documented to be involved in stem cell development (32), may repress genes during normal zinc status and produce activation on zinc depletion. Reciprocal expression of zinc-responsive genes, including zinc transporters, that maintain zinc homeostasis may be regulated by these and other transcription factors that provide opposite responses to zinc status.
The second regulatory role executed by zinc is as a regulator of cell signaling pathways. This places Zn2+ in an intracellular role that is analogous to calcium (Ca2+), except at a finer level of control. A primary mode of action is through regulation of kinase and phosphorylase activity (33, 34, 35). Zn2+ is a powerful inhibitor of phosphatases in the low micromolar range. Such control of phosphorylation and dephosphorylation could explain many of the effects attributed to zinc on activity of phosphorylated transcription factors, cell surface receptor binding of growth factors and cytokines, and activity of key phosphorylated substrates within cells. The profound effects of zinc on the immune system may thus be traceable to effects of Zn2+ availability to indirectly regulate transcription factors such as the STATs, NFAT and CREBP, as well as the phosphatase, calcineurin and the cytoplasmic protein tyrosine kinase, lymphocytespecific protein tyrosine kinase. The coordination of such activities may relate to any one or several of the 24 zinc transporters that exhibit differing specificity of expression in various cell types. Relevant examples are the influence of ZIP8 on interferon gamma (IFN-γ) production by T cells, and regulation by ZIP6 of lipopolysaccharide-induced histocompatibility complex in dendritic cells (36, 37).
Zinc is abundant in the central nervous system. A considerable portion is in the form of ionic Zn2+, in concentrations referred to as [Zn2+]i from the picomolar up to the millimolar range in synaptic vesicles (38). Zinc affects activity of N-methyl-D-aspartate and γ-aminobutyric acid receptors to influence synaptic transmission.
Neuronal [Zn] is tightly controlled by a brain-specific MT and members of both the ZnT and ZIP transporter families. The mechanism of zinc transport across the blood-brain barrier has not been established. Dietary zinc deficiency has been shown to alter brain zinc homeostasis (39, 40, 41). Cerebral ischemia leads to Zn2+ release, which participates in activation of downstream signaling cascades (particularly P13K/Akt) and oxidative and nitrosative stress that leads to necrotic, apoptotic, and autophagic death of neuronal and glial cells (38, 42).
BIOAVAILABILITY
The bioavailability of zinc is the fraction of zinc intake that is absorbed, retained, and used for physiologic functions. Zinc bioavailability in healthy individuals is determined primarily by the quantities of zinc and phytate in the diet (43). In general, the amount of zinc absorbed increases with the amount ingested, and the fractional absorption declines. However, if the diet is high in phytate, the net increase in absorbed zinc with increases in dietary intake is blunted significantly. Although changes in the quantity of bioavailable zinc directly influence the amount of zinc that is absorbed, the effect of zinc status over time on zinc absorption is questionable and, at most, plays a minor role in the maintenance of zinc homeostasis (44, 45).
Factors Affecting Bioavailability
Zinc absorption is most efficient from aqueous sources in the absence of food. With intakes lower than 5 mg, absorption is close to 100% when zinc is consumed in an aqueous solution in the fasting state (46). When zinc is ingested with food, the amount absorbed can vary from 5% to more than 50%, depending on the amount of zinc and phytate in the diet (44). Modeling studies showed that the maximal amount of zinc absorbed per day is approximately 7 mg if the diet is low in phytate and if zinc intakes are distributed throughout the day.
The efficiency of zinc absorption from plant foods is lower than that from animal source foods (43). Phytate (myoinositol hexaphosphate), which is present in plant products, especially cereals and legumes, irreversibly binds zinc in the intestinal lumen and accounts for the lower efficiency of absorption from plant foods. The negative effect on absorption is determined by the inositol hexaphosphates and pentaphosphates that bind zinc. When plant foods are fermented (leavened breads and porridges from fermented cereals), the fermenting organisms produce phytases that break down phytate and release the zinc, thus making it available for absorption (47). Because phytate is a major inhibitor of zinc absorption, the phytate-zinc molar ratio is used to estimate the likely absorption of zinc from a mixed diet. It can be calculated as follows: (phytate content of foods/660)/(zinc content of foods/65.4), where 660 and 65.4 represent the molecular or atomic weights of phytate and zinc, respectively (48). Phytate-zinc molar ratios
greater than 15 are generally considered to result in relatively poor zinc bioavailability, those between 5 and 15 are considered to result in medium bioavailability, and those less than 5 are considered to result in good bioavailability. Most plant foods have phyate-zinc molar ratios greater than 15 (seeds and nuts, whole grain cereals, beans and lentils, and tubers). Zinc absorption from those plant foods generally is less than 1 mg/100 g of food, whereas approximately 2 to 2.5 mg zinc is absorbed from 100 g of meat.
greater than 15 are generally considered to result in relatively poor zinc bioavailability, those between 5 and 15 are considered to result in medium bioavailability, and those less than 5 are considered to result in good bioavailability. Most plant foods have phyate-zinc molar ratios greater than 15 (seeds and nuts, whole grain cereals, beans and lentils, and tubers). Zinc absorption from those plant foods generally is less than 1 mg/100 g of food, whereas approximately 2 to 2.5 mg zinc is absorbed from 100 g of meat.
A mathematical model of zinc absorption has been developed to predict the amount of zinc absorbed as a function of dietary zinc and phytate (49). This model is used to develop recommendations for the zinc fortification of flour from various cereal grains and to predict the availability of zinc from zinc-fortified cereals. Plant breeding or genetic engineering strategies that either reduce the content of inhibitors (e.g., phytate) or increase the expression of compounds that enhance zinc absorption (e.g., amino acids) have been considered to improve the bioavailability of zinc from plant foods (50).
Because calcium has the propensity to form complexes with phytate and zinc that are insoluble, investigators have proposed that the phytate-zinc molar ratio should be multiplied by the dietary calcium concentration to improve the prediction of zinc bioavailability (51). The interaction with calcium is complex, however, and the effect of calcium on the phytate-zinc interaction is not consistent. Thus, the effect of calcium is usually ignored when determining the effect of phytate on zinc absorption. Supplemental or dietary calcium without phytate has little to no effect on zinc absorption at an adequate level of intake.
Nutrient Interactions
Interactions with other divalent cations in the intestinal lumen may also influence zinc bioavailability. Isotopic tracer studies indicated that iron supplementation may interfere with zinc absorption and vice versa, but only when these supplements are provided simultaneously in aqueous solutions and in disproportionate molar doses (52). No evidence indicates interference when the supplements are delivered in nearly isosmolar amounts or with food. Some longer term studies suggested that, when iron and zinc are given together, each mineral may reduce the magnitude of the response observed with single-nutrient supplementation (53), although nutritional status is still enhanced to a considerable extent.
Less information is available regarding the interaction between zinc and copper. Shifts in the dietary zinc-copper ratios from 2:1 to 15:1 had no effect on copper absorption (54). However, some studies showed a negative effect of large-dose zinc supplements (˜50 mg/day) on indicators of copper status (55). High levels of tin and cadmium inhibit zinc absorption, but the extent to which lower, physiologic levels affect absorption in humans is unknown. The relative bioavailability of nanoparticles of zinc (oxide or phosphate) is not known, but these may see use as future food additives (56).
Food Sources
The quality of dietary zinc sources is determined by the amount and bioavailability of zinc from the foods. Organs and flesh of mammals, fowl, fish, and crustaceans are the richest food sources of zinc; and because these foods do not contain phytate, they are particularly good sources of absorbable zinc. Eggs and dairy foods also are free of phytate, but they have lower zinc concentrations than organ or flesh foods. Cereals and legumes contain a modest amount of zinc, but their high phytate content reduces the amount of zinc available. Many breakfast cereals are fortified with zinc, and they are one of the major sources of zinc in the US diet. Fruits and vegetables are low in zinc.
METABOLISM
Body Zinc
Total body zinc in adult humans is between 1.5 g (females) and 2.5 g (males), making it slightly less abundant than iron. The tissue distribution of zinc is ubiquitous, however, with some tissues (e.g., the prostate) having a curiously high overall concentration. Zinc concentrations of some tissues of an adult man are shown in Table 11.1. Eightysix percent of the total body zinc is in skeletal muscle and bone. Within organs, regional differences in zinc abundance can be striking (e.g., hippocampus of the cerebral hemispheres and β cells of the pancreas and kidney cortex). These foci of zinc concentration are believed to be functionally relevant. Approximately 95% of body zinc is intracellular, with most zinc found in the cytosol. A variable amount of cytosolic zinc may reside in vesicles. The finite amount of “free” Zn2+ in cells is a subject of some debate, but investigators agree that it is extremely low (8, 17). The high binding affinities of nucleic acids, protein thiols, and nitrogen ligands account for this low concentration of free Zn2+.
TABLE 11.1 APPROXIMATE ZINC CONTENT OF MAJOR ORGANS AND TISSUES IN A NORMAL ADULT MAN | ||||||||||||||||||||||||||||||||||||||||||||||||||||||||||||
---|---|---|---|---|---|---|---|---|---|---|---|---|---|---|---|---|---|---|---|---|---|---|---|---|---|---|---|---|---|---|---|---|---|---|---|---|---|---|---|---|---|---|---|---|---|---|---|---|---|---|---|---|---|---|---|---|---|---|---|---|
|
Zinc Transporters
Following discovery of zinc transporters, all aspects of cellular zinc metabolism have been viewed within the context of transporter gene regulation by diet and hormones or cytokines and their genetic features, including mutations and polymorphisms in these genes that dictate phenotypic consequences. Two families of zinc transporter proteins have been defined. The ZnT family (solute carrier; SLC30A) and ZIP family (SLC39A) have numerous members (reviewed in 13). The 10 mammalian ZnTs (ZnT1 to ZnT10) are believed to facilitate zinc efflux across the cell membrane or into intracellular vesicles. In contrast, the 14 mammalian ZIP transporters (ZIP1 to ZIP14) facilitate zinc influx into cells or from vesicles. Both families exhibit tissue-specific expression. Subcellular localization does not appear uniform; rather, localization may be a function of physiologic conditions and body zinc status.
Some metal-metal interactions could be explained by specific transporters exhibiting multiple ion specificity (e.g., ZIP8 with zinc and manganese and ZIP14 with zinc and non-transferrin-bound iron). Genomic databases show that some transporter genes exhibit single nucleotide polymorphisms, which may have physiologic significance. Evidence exists that some ZnT and ZIP genes exhibit either up-regulation or down-regulation in response to zinc (13) and may contribute to the tight homeostatic control of zinc. Mechanisms responsible for regulating zinc transporters include transcription factors responsive to diet or physiologic conditions, mRNA stabilization, and intracellular proteolytic mechanisms.
Some transporters have been linked to specific genetic diseases and conditions, such as ZnT2 (low zinc in breast milk); ZIP4, (acrodermatitis enteropathica, with zinc malabsorption), and ZIP13 (Ehlers-Danlos syndrome). Other transporters have undefined associations with human disease, such as ZnT8 (type 1 and 2 diabetes), ZIP1, ZIP4, ZIP6, ZIP7, ZIP10, and ZIP14 (prostate, pancreatic, colon, or breast cancers), and ZIP14 (iron overload).
Intestinal Uptake and Absorption
The nature of absorbed zinc is not clear. As the zincbinding macromolecules of food are liberated and degraded to smaller zinc-binding molecules during digestion, bioavailability is generally improved. Systemic factors that influence endogenous intestinal zinc secretion or result in poor hydrolysis of luminal constituents (e.g., pancreatic insufficiency; inflammatory bowel diseases) affect intestinal zinc absorption and retention.
Evidence from model systems suggests that zinc is probably transported as free solute (Zn2+) or as a complex that may liberate Zn2+ before or after membrane transport. The way in which zinc is liberated from complexes in the neutral pH of the intestinal lumen for transport as a free solute is not known.
Absorption occurs along the entire intestinal tract (57). Perfusion studies suggested that the human jejunum exhibits the highest rate of absorption (58). Other studies with humans and animals suggested that the duodenum is quantitatively most important to the overall mass of zinc absorbed because the duodenal lumen has the highest zinc concentration after a meal (59). Endogenous secretions, including those from the pancreas, contribute to luminal zinc abundance. Overall, the extent of absorption is a function of the collective solubility of ingested zinc. Apparent absorption averages 33% and was a factor used to compute the recommended dietary allowance (RDA) (60).
Zinc absorption takes place down a concentration gradient from a relatively high luminal concentration (micromolar range) after a meal. Kinetic measurements place the Michaelis-Menten constant (Km; affinity) for zinc uptake in the micromolar range. Maximum velocity increases during zinc-deficient conditions, a finding suggesting upregulation of zinc transport when dietary zinc consumption is low (61, 62). Zinc uptake kinetics by the small intestine show mediated (saturable) and nonmediated (passive transport) components (61, 63). The saturable component may represent the sum of zinc transporter activity of enterocytes. The apically localized transporter ZIP4 (SLC39A4) is up-regulated in murine zinc deficiency (64, 65). A mutation of human ZIP4 is responsible for the zinc malabsorption disorder, acrodermatitis enteropathica (66). Defective ZIP4 in this disease leads to zinc-responsive immune dysfunction and cognitive abnormalities (67).
Within enterocytes, two proteins, MT and the transporter ZnT7, influence transcellular zinc movement (68, 69) through zinc buffering and zinc trafficking into the Golgi complex to a secretory pathway, respectively. Both are dispensable, because ablation of these genes does not block zinc absorption. ZnT1 may be the major transporter that facilitates zinc efflux from enterocytes (70). Depending on dietary zinc status the transporters expressed in enterocytes undergo varying rates of synthesis, trafficking, endocytosis, and degradation. At higher luminal zinc concentrations, generated through consumption of a zinc supplement, the nonmediated (nonsaturable) component of zinc absorption may make a major contribution to total absorption. Albumin appears to be the major portal carrier for zinc after transfer across the basolateral surface of enterocytes (71).
Homeostatic Regulation
The gastrointestinal (GI) tract plays a primary role in maintaining a stable whole body zinc content, or homeostasis. At very low levels of ingested zinc, less than 1 mg/day, nearly all the zinc from a low-phytate diet is absorbed (72). Concomitantly, a reduction in zinc secretion into the intestinal lumen occurs through pancreatic secretions and from the intestine through transepithelial flux in the serosal to mucosal direction. Mechanisms regulating intestinal zinc secretion are not well understood. As the amount of ingested zinc increases, more zinc is absorbed, although the efficiency of zinc absorption declines, and endogenous
losses increase accordingly to maintain zinc homeostasis. In humans, changes in zinc absorption with increasing zinc intake fit best with a saturable response model (73). Between 1 and approximately 9 mg dietary zinc/day, the efficiency of zinc absorption declines from nearly 1.0 to approximately 0.4. With intakes higher than approximately 9 mg/day, or the estimated average requirement (EAR), the fractional zinc absorption declines rapidly, a decline that helps to maintain homeostasis by minimizing the absorption of excess zinc. Up-regulation and down-regulation of zinc transporters and possibly other proteins involved in the transport of zinc underlie these adjustments in the efficiency of zinc absorption with changes in intake. Adjustments in absorption appear to occur rapidly because nearly tripling the zinc intakes reduced zinc absorption efficiency within 24 hours (46). Although zinc absorption responds rapidly to changes in intake, changes in absorption with shifts in zinc status appear to be minor by comparison. Studies in experimental animals showed that absorption increases during late pregnancy and lactation (74); it may also decline with aging (75).
losses increase accordingly to maintain zinc homeostasis. In humans, changes in zinc absorption with increasing zinc intake fit best with a saturable response model (73). Between 1 and approximately 9 mg dietary zinc/day, the efficiency of zinc absorption declines from nearly 1.0 to approximately 0.4. With intakes higher than approximately 9 mg/day, or the estimated average requirement (EAR), the fractional zinc absorption declines rapidly, a decline that helps to maintain homeostasis by minimizing the absorption of excess zinc. Up-regulation and down-regulation of zinc transporters and possibly other proteins involved in the transport of zinc underlie these adjustments in the efficiency of zinc absorption with changes in intake. Adjustments in absorption appear to occur rapidly because nearly tripling the zinc intakes reduced zinc absorption efficiency within 24 hours (46). Although zinc absorption responds rapidly to changes in intake, changes in absorption with shifts in zinc status appear to be minor by comparison. Studies in experimental animals showed that absorption increases during late pregnancy and lactation (74); it may also decline with aging (75).
Zinc Turnover and Transport
Plasma zinc comprises only 0.1% of total body zinc and, depending on species, represents 20% to 30% of the zinc in whole blood. Plasma concentrations are normally maintained within strict limits (˜100 µg/dL; 15 µM), with serum concentrations slightly higher in nonhemolyzed samples. Severe human zinc depletion causes a fall in plasma zinc within approximately 2 weeks (72). With zinc intakes between 3 and 10 mg/day, plasma zinc increases and then rises very slowly until a plateau is reached with intakes between 25 and 30 mg/day (75). Plasma zinc may fluctuate by as much as 20% during a day, largely because of the effects of food ingestion. The highest values of the day occur in the morning after an overnight fast.
Plasma zinc is primarily bound to albumin (70%) (71, 76). At its normal plasma concentration of 600 µM, albumin has a molar ratio with zinc of 40:1. Zinc is easily exchanged from albumin (Kd = 7.5 M-1). α2-Macroglobulin, a protease inhibitor and carrier of growth factors, binds zinc tightly and represents most of the remaining protein-bound zinc in plasma (3). A very small amount (i.e., ˜0.01%) is complexed with amino acids, especially histidine and cystine. This non-protein-bound component could be physiologically relevant and may influence urinary zinc loss. Zinc chemistry is such that virtually none circulates in a free ionized form. The flux of zinc through the plasma compartment is approximately 130 times/day (72).
A total of 70% to 80% of the blood zinc is cellular, with the concentration of leukocytes (6 mg zinc/106 cells) greater than that of erythrocytes (1 mg zinc/106 cells) (77). Erythrocyte zinc is found mostly with carbonic anhydrase (>85%), copper-zinc superoxide dismutase, and various other proteins including MT (78). In mice, membranes of erythrocytes in peripheral blood contain zinc transporter proteins, some of which are reflective of past zinc intake (79). cDNA array analyses have shown that some leukocyte genes are very sensitive to zinc (80) and may respond to plasma zinc levels or, more likely, may reflect conditions of zinc status of progenitor cells in the bone marrow.
Kinetic data with both radioactive and stable isotopes have provided important information on zinc pool turnover in humans. Two metabolic pools (rapid [˜12.5 days] and slow [˜300 days]) have been identified (81, 82). Kinetically active tissues are liver more than pancreas, kidney, and spleen. Slow turnover is found in muscle and red blood cells, followed by bone and the nervous system. Using a kinetic model, cyclic adenosine monophosphate administration to rats appeared to alter the distribution and metabolism of zinc in the thymus, skin, spleen, intestine, and, especially, the bone marrow (83). Stable isotopes of zinc have been used to identify an exchangeable zinc pool (EZP) in humans (84). The pool represents the approximately 10% of whole body zinc that exchanges with the isotope in a 2-day period. Because most zinc in the body is bound to proteins, the size of the pool is influenced by the amount of lean body mass (85). A severe dietary zinc restriction causes the size of the pool to decrease by approximately one third. This may reflect the redistribution of this more rapidly turning over zinc to other tissues (72). General features of zinc metabolism are shown in Figures 11.1 and 11.2.
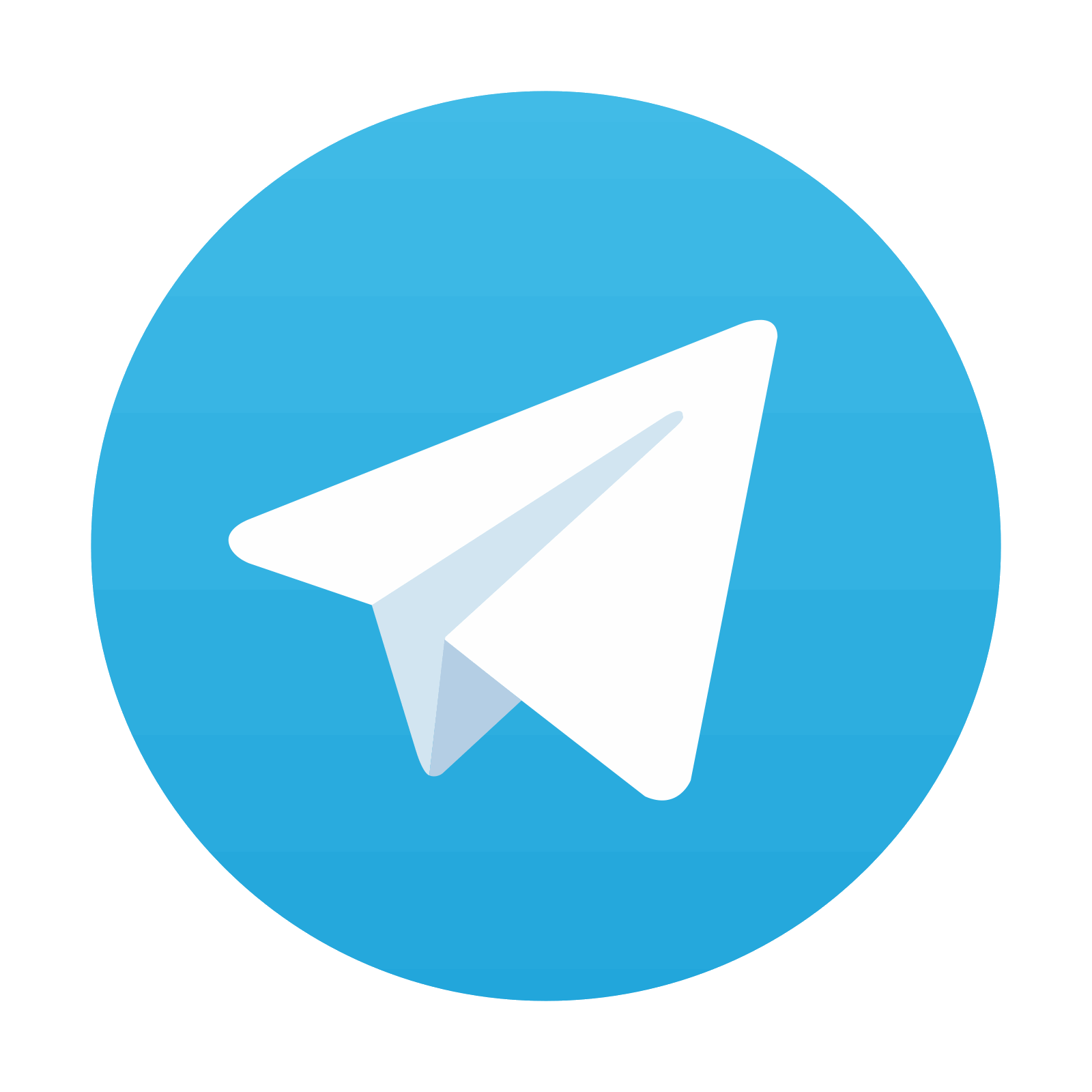
Stay updated, free articles. Join our Telegram channel
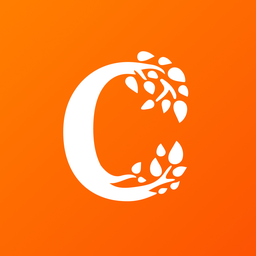
Full access? Get Clinical Tree
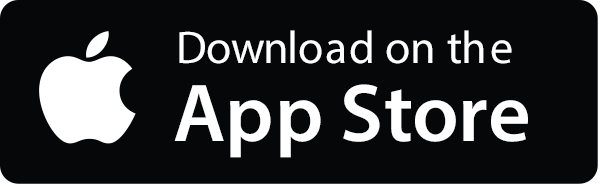
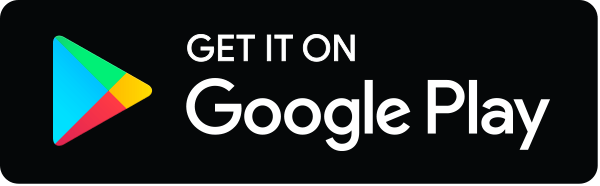