situations, calcium acts both as a signal transmitter from the outside of the cell to the inside and as an activator or stabilizer of the functional proteins involved. In fact, ionized calcium is the most common signal transmitter in all of biology. It operates from bacterial cells all the way up to cells of highly specialized tissues in higher mammals.
calcium. Part is excreted in the urine through the kidney, and part enters the slower exchange pools of soft tissue and bone. Dietary calcium influences calcium absorption and, consequently, fecal calcium and, to a lesser extent, urinary calcium excretion. An obligatory loss of calcium occurs through endogenous secretion, urine, and skin. Gender, age, and racial differences in calcium metabolism exist. Adolescents are more efficient at using calcium than are young adults, and elderly persons are the least efficient. Boys are more efficient at calcium metabolism than girls, and blacks are more efficient than whites.
TABLE 7.1 CALCIUM (Ca) METABOLISM AS INFLUENCED BY RACE AND AGE | ||||||||||||||||||||||||||||||||||||||||||||||||||||||||||||||||||||||||||||||||||||||||||
---|---|---|---|---|---|---|---|---|---|---|---|---|---|---|---|---|---|---|---|---|---|---|---|---|---|---|---|---|---|---|---|---|---|---|---|---|---|---|---|---|---|---|---|---|---|---|---|---|---|---|---|---|---|---|---|---|---|---|---|---|---|---|---|---|---|---|---|---|---|---|---|---|---|---|---|---|---|---|---|---|---|---|---|---|---|---|---|---|---|---|
|
Transcellular: This saturable (active) transfer involves a calcium-binding protein, calbindin.
Paracellular: This nonsaturable (diffusional) transfer is a linear function of calcium content of the chyme.
in young premenopausal women (25). The age-related decrease in calcium absorption from intestinal resistance to 1,25(OH)2D3 has been associated with decreased VDR levels (26), as well as with reduced estrogen levels (23).
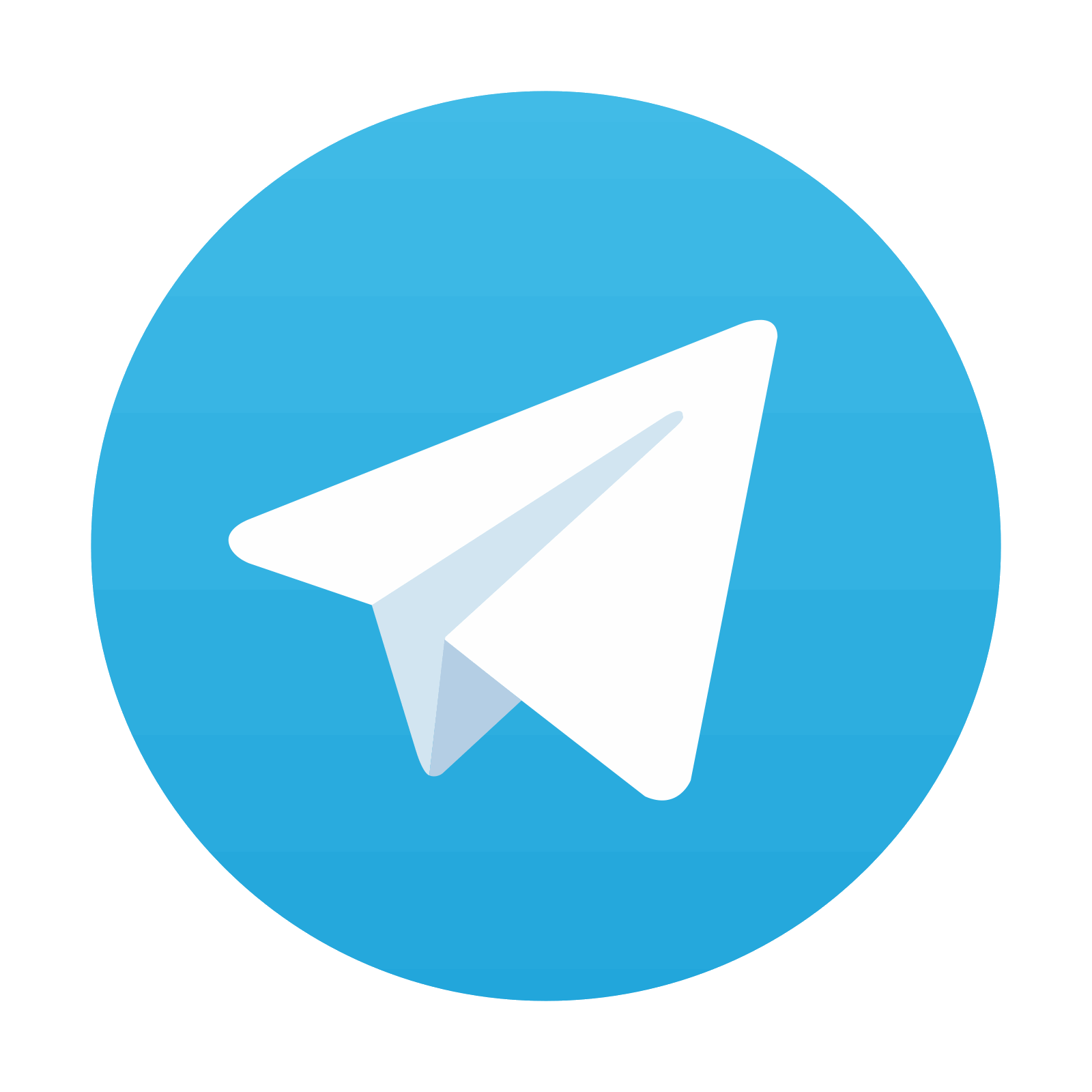
Stay updated, free articles. Join our Telegram channel
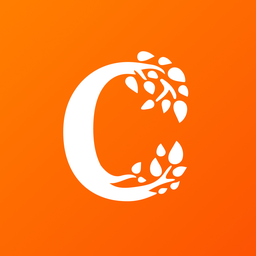
Full access? Get Clinical Tree
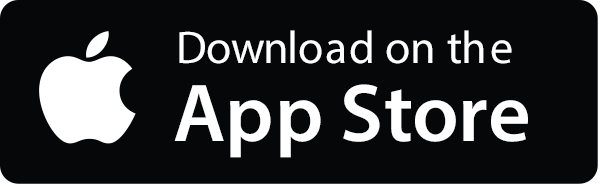
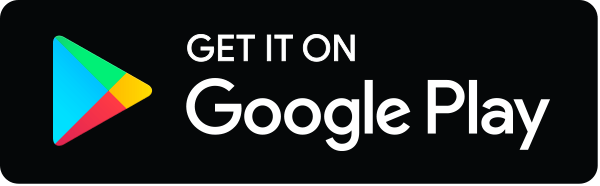