Vitamin E was discovered more than 80 years ago as a lipid-soluble substance necessary for the prevention of fetal death and resorption in rats that had been fed a rancid lard diet (Evans and Bishop, 1922). Today, it is known that vitamin E deficiency results in species-specific abnormalities, including large motor dysfunction in humans. Though the causal molecular aspects of these abnormalities remain largely unknown, all are thought to be associated with the single consensus function of vitamin E, the trapping of lipophilic free radicals. Four tocopherols and four tocotrienols occur naturally. All consist of a chromanol head group and a phytyl side chain and differ in the number and position of methyl groups on the phenol ring of the chromanol head group (Figure 29-1). The structure of a tocotrienol is similar to that of a tocopherol, except that the hydrophobic phytyl side chain of the tocotrienols contains double bonds at the 3′, 7′, and 11′ positions. In addition to these eight naturally occurring vitamers, synthetic α-tocopherol, as either free or ester forms, is available commercially. The tocopherol molecule has three chiral centers in its phytyl tail, making a total of eight stereoisomeric forms possible. The naturally occurring isomer of α-tocopherol (formerly known as D-α-tocopherol) biosynthesized by plants is the 2R, 4′R, 8′R stereoisomer, whereas synthetic α-tocopherol consists of a mixture of all eight possible stereoisomers. The tetrahedral arrangement of substituents around each asymmetric chiral center is designated R (from the Latin rectus, meaning “right”) or S (from the Latin sinister, meaning “left”). To distinguish the naturally occurring stereoisomer of α-tocopherol from the synthetic mixture, naturally occurring α-tocopherol is designated as RRR-α-tocopherol, and the synthetic mixture of α-tocopherol stereoisomers (previously known as DL-α-tocopherol) is designated as all-racemic (all-rac)-α-tocopherol. Naturally occurring tocopherols in foods exist as the free (unesterified) forms. Synthetic ester forms of α-tocopherol are produced by forming ester linkages between the 6-hydroxyl group of the phenolic ring of α-tocopherol and the carboxylate group of acetic acid (see Figure 29-1) or succinic acid. The acetate or succinate ester forms of α-tocopherol are more chemically stable and are therefore more suitable for food fortification or vitamin supplement formulations than unesterified α-tocopherol. In addition, “natural source” vitamin E preparations are produced by methylation of mixtures of tocopherols (e.g., from soybean oil), resulting in products that contain predominantly α-tocopherol. The process of intestinal absorption of vitamin E is similar to that of other lipid components of the diet. Tocopheryl esters, when present, are hydrolyzed to free tocopherol by pancreatic esterases in the proximal lumen of the small intestine. Tocopherols and tocotrienols, as constituents of bile salt micelles, are taken up, apparently by passive diffusion, into enterocytes lining the small intestine. Within the enterocyte, tocochromanols are incorporated, along with other lipids, into nascent triacylglycerol-rich chylomicrons. The chylomicrons are secreted into the intercellular space, from which they enter the lymphatics and eventually the bloodstream. Estimates of the absorption efficiency of tocopherols vary widely, but it appears that roughly half of the tocopherols consumed in foods is absorbed, with the remainder excreted in the feces. There appear to be no major differences in the rates of intestinal absorption of the various forms of vitamin E (Kayden and Traber, 1993). Because of its virtual insolubility in water, vitamin E requires special transport mechanisms in the aqueous milieu of the body. As described, dietary tocopherols taken up by the small intestine are incorporated into triacylglycerol-rich chylomicrons, secreted into the intestinal lymph, and eventually delivered to the liver in the chylomicron remnants. Newly absorbed vitamin E taken up by parenchymal cells of the liver is either incorporated into nascent very-low-density lipoproteins (VLDLs) and secreted back into the bloodstream or metabolized within the liver to water-soluble metabolites. Endogenous vitamin E in other tissues appears to be released to high-density lipoproteins (HDLs) and other plasma lipoproteins and recirculated to the liver or other tissues. Tocopherols undergo exchange between lipoproteins, facilitated by a phospholipid transfer protein found in plasma (Kostner et al., 1995). Therefore, in the fasting state, tocopherols are approximately equally distributed between low-density lipoproteins (LDLs) and HDLs. Tocopherols circulating in lipoproteins can be taken up by tissues via various receptor-mediated processes. Chylomicron remnants are taken up by the parenchymal cells of the liver via an apolipoprotein E–mediated mechanism, and LDL particles are taken up by liver and other tissues via apolipoprotein B100–mediated processes. Other mechanisms of cell uptake may involve selective uptake from HDLs via scavenger receptors, as is the case with cholesterol. In addition, some of the vitamin E in association with chylomicrons and VLDLs may be transferred to peripheral cells during the lipolysis of these triacylglycerol-rich lipoproteins by lipoprotein lipase. Studies of vitamin E delivery to tissues in transgenic mice overexpressing human lipoprotein lipase in muscle demonstrated enhanced vitamin E uptake by skeletal muscle but not by adipose tissue or brain (Sattler et al., 1996). (See Chapter 17 for an overview of lipoprotein metabolism.) The process of secretion of α-tocopherol from the liver via VLDLs is crucial to maintaining normal plasma vitamin E levels. A tocopherol-binding protein, called α-tocopherol transfer protein (α-TTP) plays an essential role in this process. This soluble protein is found predominantly in liver, and preferentially binds RRR-α-tocopherol relative to other forms of vitamin E (Manor and Morley, 2008). It is only one of two proteins known to bind vitamin E with high affinity; the other is cytochrome P450-4F2, as discussed later. α-TTP exhibits two unique properties: high affinity binding of α-tocopherol, and transfer of α-tocopherol from one membrane to another in a process involving direct membrane interaction (Morley et al., 2008). In the liver, α-TTP facilitates the secretion of α-tocopherol into the plasma lipoprotein pool (Traber et al., 1993) via a non–Golgi-dependent mechanism that has not been fully elucidated (Kaempf-Rotzoll et al., 2003). α-TTP has been reported to associate with lysosomes or late endosomes, where it presumably binds and transfers predominantly α-tocopherol to another vesicular compartment for translocation to the cell surface. The ATP-binding cassette transporter A1 (ABCA1), which lipidates apoA1 and lipid-poor HDL with phospholipid and cholesterol, has been reported to facilitate the secretion of vitamin E from cells (Oram et al., 2001; Qian et al., 2005), but a physical interaction between α-TTP and ABCA1 in the liver has yet to be demonstrated. This process contributes to the preferential enrichment of LDLs and HDLs with α-tocopherol compared with the other forms of vitamin E. A critical role for α-TTP in maintaining normal plasma tocopherol concentration has been demonstrated in patients with familial ataxia with isolated vitamin E deficiency, or AVED (Gotoda et al., 1995; Ouahchi et al., 1995; Kayden and Traber, 1993). These patients have clear signs of vitamin E deficiency (extremely low plasma vitamin E and neurological abnormalities) but have no fat malabsorption or lipoprotein abnormalities. Absence of functional α-TTP in these patients impairs secretion of α-tocopherol from liver into the bloodstream, resulting in very low concentrations of plasma vitamin E. Presumably the α-tocopherol not secreted into the bloodstream is secreted into the bile. The plasma vitamin E level of AVED patients can be normalized with high-dose vitamin E supplementation. Tocopherols and tocotrienols other than α-tocopherol undergo extensive postabsorptive metabolism to water-soluble metabolites that are excreted primarily in the urine. This catabolic process involves severe truncation of the hydrophobic phytyl side chain, the moiety responsible for the fat-solubility of vitamin E. The metabolic pathway is depicted in Figure 29-2 and involves an initial hydroxylation of a terminal methyl group (ω-hydroxylation) of the phytyl side chain. In human beings, the enzyme cytochrome P450-4F2 (CYP4F2), an endoplasmic reticulum enzyme that requires NADPH and is expressed predominantly in the liver, has been implicated in this reaction (Sontag and Parker, 2002). The hydroxylated intermediate is further oxidized, apparently by an NAD-dependent dehydrogenase, to the corresponding ω-carboxychromanol. Truncation of the phytyl side chain subsequently occurs by sequential removal of two- or three-carbon units, ultimately yielding the 3′-carboxyethylhydroxychromanol (CEHC) (see Figure 29-2). Despite the relatively high water solubility of these short-chain metabolites, they appear to be largely conjugated with glucuronic acid by the action of uridine 5′-diphospho-glucuronosyltransferase, presumably at the phenolic hydroxyl group, to further facilitate their excretion in urine. α-Tocopherol is a relatively poor substrate for this catabolic pathway, whereas other tocopherols and the tocotrienols are good substrates (Sontag and Parker, 2007). Therefore the tocopherol-ω-oxidation pathway is considered to play a central role in the selective tissue deposition of α-tocopherol via the preferential elimination of the other forms of vitamin E, resulting in the “α-tocopherol phenotype.” This phenotype is widely expressed in the animal kingdom. Though α-TTP also contributes to this phenotype (Figure 29-3), it does not appear to be essential, because the fruit fly, Drosophila, expresses the α-tocopherol phenotype and a cytochrome P450–mediated ω-oxidation pathway similar to that of mammals, yet does not express a protein with the hallmark activity of α-TTP (Parker and McCormick, 2005). To date ω-oxidation is the only known pathway of vitamin E metabolism. Usual plasma vitamin E concentrations in humans range from 20 to 30 μmol of α-tocopherol per liter or about 5 to 8 mmol α-tocopherol per mole of cholesterol. Plasma concentrations of γ-tocopherol are typically about one tenth those of α-tocopherol, and smaller amounts of δ-tocopherol are usually present. The relative proportions of tocopherols in plasma (and tissues) lie in stark contrast to those found in foods, as dietary intakes of γ-tocopherol, and occasionally even δ-tocopherol, exceed those of α-tocopherol. This difference, manifested as the “α-tocopherol phenotype,” is brought about by the actions of α-TTP and the tocopherol-ω-oxidation pathway described in Figure 29-3. With respect to α-tocopherol, plasma concentrations appear to be linearly related to intake over the range of intakes possible from foods (i.e., up to approximately 20 mg/day). At greater intakes (from supplements) plasma concentrations are nonlinearly related to intake such that only relatively small increases in plasma α-tocopherol are achieved even with a tenfold increase in intake over that from diet alone. A supplemental intake of α-tocopherol of roughly 400 mg/day is usually needed to achieve a doubling of the plasma α-tocopherol concentration, and the maximal plasma α-tocopherol concentration achievable is roughly 50 to 60 μmol/L (Princen et al., 1995). The reason for this nonlinearity at high intakes of α-tocopherol is not clear. A consequence of supplementation with α-tocopherol is suppression of the plasma concentrations of γ- and δ-tocopherols. The mechanism responsible for this suppression is not known. Supplementation with γ-tocopherol does not suppress concentrations of α-tocopherol. Because tocopherols are transported by plasma lipoproteins, individuals with higher plasma cholesterol levels typically have higher plasma tocopherol concentrations. In disorders causing lipid malabsorption (such as in individuals with cystic fibrosis or abetalipoproteinemia), plasma lipid, lipoprotein, and vitamin E concentrations frequently are all reduced concurrently (Machlin, 1991). The tocopherols and tocotrienols have long been recognized to be superior free radical antioxidants when tested in vitro, and it is this characteristic that is most often ascribed to their essentiality in vivo. Vitamin E (primarily α-tocopherol) is the major lipid-soluble, free radical chain-breaking antioxidant found in plasma, red blood cells, and tissues, and it plays an essential role in maintaining the integrity of biological membranes (Burton and Traber, 1990). Vitamin E is present in all cellular membranes, where it is thought to act to protect membrane lipids and perhaps proteins from free radical–induced oxidative damage. The reaction of α-tocopherol with polyunsaturated fatty acid peroxyl radicals to prevent uncontrolled lipid peroxidation is the best understood action of vitamin E. A variety of carbon- and oxygen-centered free radicals are generated during the course of normal metabolism in vivo. These include the superoxide, lipid alkoxyl, and peroxyl radicals. Among them, peroxyl radicals derived from polyunsaturated fatty acids have special significance because of their involvement in lipid peroxidation, which is the most common indicator of free radical production in living systems. (See Chapter 18 for more information on polyunsaturated fatty acids and lipid peroxidation.)
Vitamin E
Nomenclature and Structure of Vitamin E
Absorption, Transport, and Metabolism of Vitamin E
Absorption
Plasma Transport and Tissue Uptake
Hepatic Secretion and the Role of α-Tocopherol Transfer Protein
Metabolism of Vitamin E and the Role of CYTOCHROME P450-4F2
Plasma Concentration of Vitamin E and Relationship with Dietary Intake
Biological Functions of Vitamin E
Vitamin E as a Free Radical–Scavenging Antioxidant
(Reaction I)
Stay updated, free articles. Join our Telegram channel
Full access? Get Clinical Tree
Vitamin E
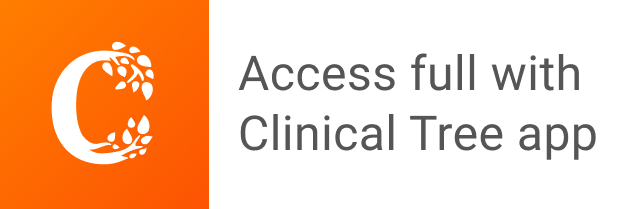