Vitamin A was initially recognized as an essential growth factor present in foods of animal origin such as animal fats and fish oils, and this factor was called fat-soluble A (McCollum and Davis, 1913; Osborne and Mendel, 1919). It was also observed that some plants display an activity similar to this fat-soluble A factor. Subsequently, in the early 1930s it became clear that plant-derived compounds, known as carotenoids, are precursors for vitamin A and can be converted to retinol in animals. The structures of some physiologically important retinoids as well as the most active provitamin A carotenoid, all-trans-β-carotene, are shown in Figure 30-1. Retinoids are composed of three distinct structural domains: a β-ionone ring, a spacer of a polyunsaturated chain, and a polar end-group. The polar end-group of naturally occurring retinoids can exist at several oxidation states varying from the low oxidation state of retinol, to retinal, and to the even higher oxidation state in retinoic acid (RA). Vitamin A is stored in vivo in the form of retinyl esters in which the retinyl moiety is esterified with a long-chain fatty acid with concomitant loss of the polar end-group (see Figure 30-1). Retinol can also be converted in vivo to conjugated species with larger, more polar, end-groups (e.g., retinoyl β-glucuronide; see Figure 30-1). The process of the visual signal transduction is a classical example of a G protein–mediated signaling cascade and is well characterized (Figure 30-2) (Wald, 1968; Travis et al., 2007; von Lintig et al., 2010). Absorption of a photon by rhodopsin-bound 11-cis-retinal results in isomerization of the chromophore to the all-trans form, a process that induces the protein to undergo several conformational changes through a series of short-lived intermediates. One of the protein intermediates (metarhodopsin II, R∗ in Figure 30-2) interacts with another membrane protein named transducin. Transducin is a G protein; its interaction with R∗ leads to an exchange of a transducin-bound guanosine diphosphate (GDP) for a guanosine triphosphate (GTP). In the GTP-bound (activated) state, transducin activates an enzyme called phosphodiesterase. Phosphodiesterase catalyzes the breakdown of cyclic guanosine monophosphate (cGMP), which keeps sodium channels in the plasma membranes of rod outer segments in the open state, to an inactive product, GMP. Because the level of cGMP in rod outer segments in the dark is high (~0.07 mM), the sodium channels are open and the membranes of the cells are depolarized. Activation of phosphodiesterase following illumination results in lower levels of cGMP and leads to closing of the sodium channels and to hyperpolarization of the plasma membrane. The process of visual transduction is regulated further at several levels, including phosphorylation of rhodopsin intermediates, enzymatic hydrolysis of retinal from metarhodopsin II, and termination of the interaction between activated rhodopsin and transducin by the protein arrestin. Bleached rhodopsin can be regenerated in the dark by 11-cis-retinal freshly supplied to the photoreceptors from the adjacent retinal pigment epithelium (RPE) cells (Figure 30-3), which take up vitamin A from blood and store it in the form of all-trans-retinyl esters. These storage species are enzymatically converted in RPE cells to 11-cis-retinal, which is then transported across the interphotoreceptor matrix to photoreceptors. The metabolism and transport of retinoids in the eye are discussed later in this chapter. Retinoids have profound effects on the differentiation and growth of a variety of normal and neoplastically transformed cells. One striking example is the differentiation pattern of HL-60 cells, which originated from a human promyelocytic leukemia. These cells differentiate into macrophages when treated with 1,25-dihydroxyvitamin D3 or with phorbol esters. In contrast, when treated with RA, HL-60 cells differentiate into granulocytes, and this is followed by an arrest in cell proliferation. Other examples include the ability of RA to enhance neuronal differentiation or, in contrast, to inhibit the differentiation of fibroblasts into adipocytes. Retinoids also control the formation of particular patterns, such as digit development, during embryogenesis (Hoffman and Eichele, 1994). In addition, studies of isolated cells, animal models, and humans have demonstrated that retinoids can inhibit cancer development and, in some cases, induce transformed cells to revert to a normal phenotype. Indeed, RA is successfully used in treatment of human acute promyelocytic leukemia and other cancers (Soprano et al., 2004). Retinoic acids regulate gene expression by activating specific transcription factors, termed retinoid receptors, which are members of a superfamily of the nuclear hormone receptor ligand-inducible transcription factors. Retinoid receptors bind to DNA recognition sequences (response elements) in regulatory regions of target genes, and upon binding of RA, facilitate the rate of transcription of these genes (Germain et al., 2006a, 2006b). Like other nuclear hormone receptors, retinoid receptors are composed of several functional domains (Figure 30-4). The N-terminal region of the receptors (A/B domain) which contains a basal, or ligand-independent, activation function termed AF-1. The DNA-binding domain (domain C) contains zinc-fingers responsible for the association of the receptor with DNA (see Chapter 37 for more information on the role of zinc finger motifs in DNA binding by proteins). Domain D is a hinge region that confers flexibility to the protein molecule. Domain E, termed the ligand binding domain, contains the ligand-binding pocket and is responsible for ligand-induced transcriptional activation by the receptors. The ligand-binding domain also contains regions that mediate the interactions of retinoid receptors with a variety of other proteins (see subsequent text). The C-terminal region, which is present in some but not all nuclear receptors, is termed the F domain, and its function is unknown at present. All-trans-RA enhances the transcription of target genes by activating three RA receptors (RARα, RARβ, and RARγ) (Germain et al., 2006a) and a nuclear receptor termed peroxisome proliferator–activated receptor β/δ (PPARβ/δ) (Schug et al., 2007). The partitioning of the all-trans-RA hormone between these receptors is regulated by specific RA-binding proteins that selectively deliver it to particular receptors (see later). RARs and PPARβ/δ control the expression of a distinct array of target genes. As the binding proteins determine whether the hormone will be targeted to RAR or PPARβ/δ in a specific cell, cellular responses to RA vary depending on the relative expression levels of these proteins. A third type of retinoid receptors, termed the retinoid X receptors (RXRα, RXRβ, RXRγ), can be activated by the 9-cis-isomer of RA (9cRA). However, 9cRA is not found in all tissues that express RXR and it is uncertain whether this compound serves as the physiological ligand for this receptor (Calleja et al., 2006; Germain et al., 2006b). Like other nuclear hormone receptors, RAR, PPARβ/δ, and RXR bind to their DNA response elements as dimers. Dimerization, which is stabilized by strong interactions between the ligand-binding domains and by weaker interactions between the DNA-binding domains of the two monomers, serves to increase the specificity of binding of receptors to particular DNA sequences as well as the strength of their interactions with response elements. Mirroring dimer formation by the proteins, DNA response elements for retinoid receptors are usually arranged as two direct repeats of the same hexanucleotide sequence. RAR and PPARβ/δ homodimers do not readily form. Instead, these receptors associate with RXR with a high affinity to form heterodimers, and these RXR-RAR and RXR-PPAR heterodimers serve as the transcriptionally active species (Durand et al., 1992; Green and Wahli, 1994). In addition to heterodimerization with RAR and PPARβ/δ, RXRs can also interact with other members of the hormone receptor family. For example, they can form heterodimers with the vitamin D3 receptor (VDR); the thyroid hormone receptor (TR); the peroxisome proliferator–activated receptors PPARα and PPARγ, which are activated by long-chain fatty acids and some of their metabolites; and with liver X receptor (LXR), which responds to cholesterol metabolites (Figure 30-5; also see Chapters 18 and 31 for more information on transcriptional control by fatty acids and vitamin D). In addition, RXR can bind to DNA and regulate the transcription of target genes as a homodimer (RXR-RXR). RXR has also been reported to form active heterodimers with some orphan nuclear receptors (i.e., proteins that belong to the superfamily of nuclear receptors but for which the ligand is unknown). Heterodimers of RXR with other nuclear receptors can respond to the individual ligands of the two partners and consequently the transcriptional activities of RXR-heterodimers are regulated by more than one type of ligand. RXRs thus function as “master regulators” of several signaling nutrients and hormones as they converge at the genome to regulate gene expression (see Figure 30-5). Modulation of gene transcription by nuclear receptors depends critically on binding of activating ligands, which controls the association of the receptors with coregulatory proteins (Hsia et al., 2010) (Figure 30-6). In the absence of ligands, nuclear receptors associate with proteins that function as transcriptional corepressors. These proteins display enzymatic activities that catalyze deacetylation of histones, resulting in a more compact chromatin structure that leads to repression of transcriptional rates. Upon ligand binding, nuclear receptors undergo a conformational change (Moras and Gronemeyer, 1998) that leads to dissociation of corepressors and recruitment of transcriptional coactivators. Some coactivators catalyze histone acetylation, thereby loosening the structure of the chromatin (Hsia et al., 2010), whereas others, such as components of the Mediator complex, interact with the general transcription machinery and stabilize the recruitment of RNA polymerase II to the target gene promoter (Ito and Roeder, 2001; Rachez and Freedman, 2001). Ligand-activated receptors thus facilitate the transcription of their target genes. Unlike other receptors, including RAR and PPARβ/δ, the association of RXR with corepressors is weak, suggesting that ligand-dependent activation of this receptor might operate via a different mechanism. Indeed, it was demonstrated that RXR is unique in that, in the absence of its ligand, it exists as a transcriptionally silent homotetramer and that ligand binding results in rapid dissociation of RXR tetramers to the active dimeric species (Kersten et al., 1995, 1998; Gampe et al., 2000). Ligand-induced dissociation of RXR tetramers thus seems to be the first step in activation of this unusual receptor. In addition to retinal and RA, other retinoids are endogenously present in a variety of tissues. The functions of these derivatives are not completely understood but some of them have been shown to be biologically active. For example, retinol itself as well as the metabolite 14-hydroxy-retro-retinol (see Figure 30-1), have been implicated in regulating lymphocyte physiology (Ross and Hammerling, 1994; Chiu et al., 2008). The mechanisms by which these compounds support lymphocyte growth are incompletely understood. They do not associate with any of the known nuclear retinoid receptors and may function by other signaling pathways. Another biologically active retinoid is 3,4-didehydroretinol, also known as vitamin A2. It is abundant in freshwater fish, where its metabolite 11-cis-dehydroretinal can serve as a ligand for visual pigments. In humans, 3,4-didehydroretinol was reported to accumulate in tissues of individuals with psoriasis and several other disorders of keratinization (Vahlquist and Torma, 1988). 3,4-Didehydroretinoic acid was found in chick limb buds, where it can affect development, presumably by activating retinoid nuclear receptors (Thaller and Eichele, 1990). It was reported that oxidized retinoid metabolites such as 4-oxo-RA avidly bind to RARβ and are important in determining the positions at which particular digits develop in early embryos (Pijnappel et al., 1993). In addition, it has been demonstrated that some proteins are modified by covalent retinoylation (Takahashi and Breitman, 1991). At present, the effects of retinoylation on the functions of proteins modified in this fashion are not known. Two major forms of vitamin A are present in the diet: retinyl esters, which are derived from animal sources, and carotenoids, mainly β-carotene, which originate from plants (see Figure 30-1). Retinyl esters are hydrolyzed in the intestinal lumen to yield free retinol and the corresponding fatty acid (Figure 30-7). Retinyl ester hydrolysis requires the presence of bile salts that serve to solubilize the retinyl esters in mixed micelles and to activate the hydrolyzing enzymes. Several enzymes that are present in the intestinal lumen may be involved in the hydrolysis of dietary retinyl esters. Carboxylester lipase is secreted into the intestinal lumen from the pancreas and has been shown in vitro to display retinyl ester hydrolase activity. In addition, a retinyl ester hydrolase that is intrinsic to the brush border membrane of the small intestine has been characterized in rats and humans (Rigtrup and Ong, 1992). The different hydrolyzing enzymes are activated by different types of bile salts and have distinct substrate specificities. For example, whereas the pancreatic carboxylester lipase is selective for short-chain retinyl esters, the brush border membrane enzyme preferentially hydrolyzes retinyl esters containing a long-chain fatty acid such as palmitate or stearate. Following hydrolysis, retinol diffuses into the enterocytes in a concentration-dependent manner. In contrast, uptake of carotenoids is mediated by transporters (During and Harrison, 2007). Absorbed β-carotene is centrally cleaved into two molecules of retinal by β-carotene 15,15′-monooxygenase (BCMO1) (Figure 30-8). This enzyme is most highly expressed in the intestinal mucosa but is also found in liver, kidney, lungs, retina, and the brain. In addition, activities that catalyze eccentric cleavage have been reported. The amounts of carotenoids that can pass intact from intestinal cells into blood vary considerably between different species. In the rat, very limited amounts of carotenoids pass into the circulation. In humans, 60% to 70% of absorbed β-carotene is cleaved in the intestine, with the remainder transferred intact into blood and deposited in several tissues such as liver and adipose tissue. The serum level of carotenoids reflects dietary intake, suggesting that a significant fraction of newly absorbed carotenoids are exported from enterocytes into blood without being metabolically converted within these cells. Vitamin A is transported in chylomicrons and stored in the liver in the form of retinyl esters in which retinol is esterified with a long-chain fatty acid. Esterification is accompanied by loss of the polar end-groups of both the retinyl and the fatty acyl moieties and results in exceedingly hydrophobic species, which accumulate within lipid droplets in storage cells. Two classes of enzymes that can catalyze the formation of retinyl esters have been identified (Blomhoff and Blomhoff, 2006; Moise et al., 2007). One of these uses activated fatty acids in the form of fatty acyl-CoAs and is termed acyl coenzyme A:retinol acyltransferase (ARAT) (Figure 30-9). A second type of retinol-esterifying enzyme that functions independently of the presence of exogenous fatty acyl-CoAs is known as lecithin:retinol acyltransferase (LRAT) (Figure 30-10). This enzyme synthesizes retinyl esters by catalyzing the transesterification of a fatty acyl moiety from the sn-1 position of phosphatidylcholine to retinol. Both ARAT and LRAT are integral membrane proteins and are associated with the microsomal (endoplasmic reticulum) fractions of cells of various tissues. Retinyl esters in plasma and in the liver mainly contain the fatty acyl moieties of palmitate and stearate, regardless of the composition of fatty acids in the diet. The composition of the acyl chains in retinyl esters thus corresponds to the primary species of fatty acids found in the sn-1 position of phosphatidylcholines, implicating LRAT as the predominant enzyme in esterification of retinol in the intestine and liver. LRAT messenger RNA (mRNA) is present in intestines, liver, testes, retina, and other tissues known for high activities of vitamin A processing. It is worth noting, however, that it has also been reported that retinol esterification in lactating mammary gland is catalyzed mainly by ARAT (Randolph et al., 1991). Hence the relative contributions of the two enzymes to retinyl ester synthesis may be tissue-specific. The major site of vitamin A storage in the body is the liver. It has been reported that retinyl esters in chylomicrons may be hydrolyzed by a lipoprotein lipase at the adipocyte surface, and it was suggested that this activity facilitates uptake of retinol by these cells (Blaner et al., 1994). However, a significant fraction of retinyl esters is retained in chylomicron remnants, and these are cleared from plasma into liver parenchymal cells by receptor-mediated endocytosis. Following uptake of retinyl esters from the circulation by hepatic parenchymal cells, vitamin A is transferred to hepatic stellate cells where it is stored. Although the mechanism by which vitamin A is transported between the two cell types is not completely understood, it has been shown that chylomicron retinyl esters are hydrolyzed in the parenchymal cells and that new retinyl esters are formed in the stellate cells. This suggests that vitamin A is transported between the two cell types in the form of free retinol (Blomhoff and Blomhoff, 2006). Under normal dietary conditions, the main fraction of retinyl esters in the liver is found in the stellate cells where they accumulate in lipid droplets. In vitamin A–deficient animals, retinoids are mobilized from the stellate cells into parenchymal cells in a process that, again, seems to involve hydrolysis of retinyl esters. Therefore absorption and mobilization of vitamin A between different tissues and cells seems to require continuous hydrolysis and re-formation of retinyl esters (Figure 30-11). Two classes of enzymes can function as retinol dehydrogenases in vitro: (1) cytosolic medium-chain alcohol dehydrogenases, and (2) members of the family of short-chain dehydrogenases/reductases (SDRs) that are associated with the membranes of the endoplasmic reticulum of various cells. In contrast to soluble alcohol dehydrogenases, it has been reported that some SDR-type retinol dehydrogenases are able to metabolize retinol when bound to the cellular retinol-binding protein (CRBP). Hence while the relative contributions of soluble versus microsomal activities to retinal synthesis in various tissues have not been completely established, it is currently believed that retinal formation in vivo occurs mainly by microsomal SDRs. The first such enzymes to be cloned were the hepatic all-trans-retinol dehydrogenase and the cis-retinol dehydrogenase that is highly expressed in the retinal pigment epithelium of the eye (Chai et al., 1995; Simon et al., 1996). Subsequently, multiple isozymes have been identified in various tissues. Some of these display a dual specificity toward cis– and all-trans-retinol, whereas others are more selective for particular isomeric configurations. Several mammalian cytosolic retinal dehydrogenases (RalDHs) that catalyze the NAD+-dependent oxidation of all-trans-retinal to all-trans-RA have been identified. Recent studies using genetically manipulated mouse models indicated that these enzymes indeed play critical roles in RA synthesis in vivo. It was shown that genetic ablation of RalDH2 results in lethality on about embryonic day 9.5 because of severe trunk, hindbrain, and heart defects resembling those of vitamin A–deficient embryos (Niederreither et al., 1999). Homozygous RalDH1 knockout mice are viable and exhibit no gross malformations. However, it has been reported that RA synthesis in liver of these mice is greatly reduced, suggesting that RalDH1 participates in RA synthesis in vivo (Fan et al., 2003). Mice in which RalDH3 has been genetically knocked out display suppressed RA synthesis and ocular and nasal malformations similar to those observed in vitamin A–deficient fetuses (Dupe et al., 2003). These defects can be prevented by maternal treatment with RA, demonstrating the importance of the enzyme in RA synthesis. Less is known about the physiological function of RalDH4, which appears to display selectivity toward 9-cis-retinal, suggesting that it may play a role in synthesis of 9-cis-RA (Lin et al., 2003).
Vitamin A
Chemistry and Physical Properties of Vitamin a and Carotenoids
Lability and Limited Solubility of Retinoids in Water
Physiological Functions of Vitamin A
Role of 11-cis-Retinal in Vision
Regulation of Cell Proliferation and Differentiation by Retinoic Acids
ACTIVATION OF Retinoid Nuclear Receptors
Structure of Retinoid Nuclear Receptors
Types of Retinoid Receptors: RAR, PPARβ/δ), and RXR
RAR and PPARβ/δ Heterodimers
RXR Homo- and Heterodimers
Role of Coregulatory Proteins
Other Retinoids and Their Functions
Absorption, Transport, Storage, and Metabolism of Vitamin a and Carotenoids
Absorption and Metabolism of Vitamin a in the Intestines
Esterification of Retinol by ARAT And LRAT
Esterification of Retinol in the Intestine
Delivery of Retinyl Esters to the Liver
Hydrolysis and Re-formation of Retinyl Esters
Synthesis of Retinal and Retinoic Acid From Retinol
Retinol Dehydrogenases
Retinal Dehydrogenases
Vitamin A
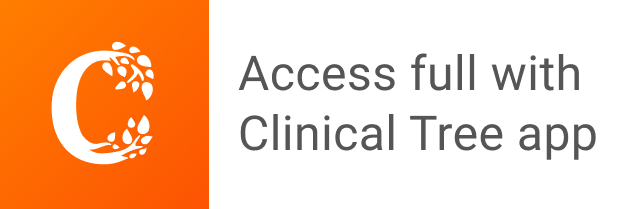