Fig. 3.1
(a) Infant admitted in a Neonatal Intensive Care Unit for a severe form of NEC with multiorgan failure. (b) Abdominal radiograph of a neonate with NEC showing diffuse bowel dilatation and extensive intramural gas in the right lower quadrant (arrow). (c) Intestinal segments affected by NEC: patchy areas of necrosis (white arrows), edematous and dilated bowel loop (yellow arrow) with questionable tissue viability
2.4 Outcome
Despite advances in neonatal intensive care, the mortality from NEC remains high, ranging from 10 to 30 %. Up to one third of surviving children experience significant morbidity including neurodevelopmental impairment, vision and hearing impairment, failure to thrive, bowel obstruction and short bowel syndrome [13]. NEC remains the main cause of short bowel syndrome in patients born with a normal gastrointestinal tract and one of the principal causes of intestinal failure in childhood.
2.5 Current Demands
Despite the high clinical impact of NEC in the pediatric population, many questions remain to be addressed concerning its etiology, diagnosis and treatment. In clinical practice, new medical approaches able to prevent the progression of the disease and its long-term complications are of absolute need. Current research efforts are mainly focused on the one hand on unravelling the pathogenesis of NEC and on the other on developing new therapeutic strategies.
2.6 Animal Models of NEC
There are a number of accepted animal models to study NEC. They all aim to create in animals a necrotic bowel resembling that of human newborns affected by NEC. Many models induce intestinal damage through administration of pro-inflammatory cytokines (e.g. PAF and TNF-alpha); others are based on the supply of an ischemic stress to the intestine (e.g. superior mesenteric artery clamping, systemic hypoxia) [14, 15]. Very few models combine a hypoxic and inflammatory stress (e.g. increasing gut intraluminal pressure and injecting E. coli into the intestinal lumen) [16]. The majority of available models do not contain the aspect of prematurity and the multiple pathogenetic elements that are present in NEC.
Among existing experimental models, the one proposed by Barlow in 1974 is the most reliable and the one that most closely resembles NEC [17, 13] as it employs all the main contributory factors involved in the development of human disease. This model mainly consists in the administration of bacteria (i.e. Klebsiella spp.), hyperosmolar formula and hypoxic stress to newborn rats, delivered by Caesarean section, during their first 4 days of life [18]. NEC reproducibility using this model, however, varies among different studies with a prevalence ranging from 35 to 71 % [19, 20]; this is mainly due to different grades of intestinal bacterial colonization depending on microbiological variations among animal facilities. To overcome this source of variability, an LPS-modified gavage model of NEC has been established [21, 22], in which the oral administration of bacteria is substituted with that of bacterial lipopolysaccharide (LPS). This LPS-modified gavage model of NEC is consistent and reproducible with a constant NEC incidence, estimated on gut histology, of 70 % at the end of the treatment [22] (Fig. 3.2). Moreover, in this variant of the Barlow’s model two novel parameters (i.e. gut macroscopic appearance and animal clinical status) well correlate with intestinal histological damage, thus permitting to evaluate NEC severity with no need to process gut specimens for histology [22]. As intensive care support and operative treatment normally provided to human neonates with NEC (e.g. ventilation, cardiovascular support, intravenous fluids, antibiotics, parenteral nutrition) cannot be given to pup rats, this model is not compatible with long-term survival [23]. At present only inhibition of nuclear factor-kB [24] and administration of heparin-binding epidermal growth factor-like growth factor (HB-EGF) [21] have been shown, in this model, to decrease animal mortality at 72 and 96 h, respectively.


Fig. 3.2
Histological changes of the ileum in rats with NEC induced employing the LPS-modified Barlow’s model. Representative sections for each grading score (H & E staining; original magnification 200×): grade 0: normal intestine; grade 1: disarrangement of villus enterocytes and mild villus core separation; grade 2: disarrangement of villus enterocytes and severe villus core separation; grade 3: epithelial sloughing of the villi. NEC is defined as grade 2 or above
3 Stem Cell Therapy as a New Strategy for the Treatment of NEC
NEC is a devastating disease of newborns for which no specific treatment is currently available. Thus, there is the need of new therapeutic strategies capable either to repair the functioning intestinal surface or to avoid its massive destruction. Ever-increasing experimental evidences support the hypothesis that stem cells may promote tissue repair/regeneration in NEC as well as in other intestinal diseases with similar physiopathological features.
3.1 Potential of Stem Cells in Gut Damage
Due to the complexity of the gut as an organ and the variety of its cell populations, regenerative medicine for intestinal diseases is still at an early stage of development. In 1993, for the first time a case of Crohn’s disease regression was reported following haematopoietic stem cell transplantation for haematopoietic malignancy [25]. This beneficial effect was thought to be determined by the radical ablation of an aberrant immune system followed by reconstitution of a naive, non-aberrant immune compartment. By in situ hybridization of intestinal specimens of women who had received bone marrow transplant from male donors, in 2002 it was demonstrated that donor-derived cells do also integrate in the recipient intestinal tract persisting long-life after bone marrow transplantation [26].
After these first reports, the therapeutic potential of stem cells was tested in animal models of intestinal damage. On the one hand, several groups reported that bone marrow-derived mesenchymal stem cells could: integrate in the gut [27]; differentiate into intestinal epithelial cells [28, 26]; and contribute to the repair and formation of intestinal blood vessels via differentiation into myofibroblasts, endothelial cells, vascular smooth muscle cells and pericytes [29, 30]. On the other hand, different authors demonstrated that bone marrow-derived mesenchymal stem cells could reduce intestinal inflammation in experimental colitis via paracrine mechanisms (e.g. decrease of inflammatory mediators such as TNF-α, IL-1β) [31,32].
The means by which mesenchymal stem cells provide clinical benefit in animal models of intestinal damage is still under investigation. Available evidences support the hypothesis that stem cells could act both by regenerative and immunoregulatory mechanisms: stem cells can directly repopulate the intestine via differentiation into committed cells, and can reduce inflammation via paracrine mechanisms [33].
3.2 Stem Cells as a Treatment of Sepsis
There is a growing body of evidence that stem cells may offer therapeutic benefit in sepsis. In 2007, Xu et al. demonstrated that mesenchymal stem cell administration in mice subjected to intraperitoneal administration of lipopolysaccharide (LPS) resulted in a significant decrease of pro-inflammatory cytokines such as IFN-γ, IL-1β and IL-6 [34]. Gupta et al. showed that the intrapulmonary delivery of mesenchymal stem cells in LPS-inoculated mice resulted in less pulmonary oedema, decreased inflammatory cytokines in plasma and bronchoalveolar lavage, and increased survival [35]. Nemeth et al. demonstrated that administration of mesenchymal stem cells in mice before or shortly after inducing sepsis by coecal ligation and puncture reduced mortality and improved organ function [36]. The authors found that mesenchymal stem cells, activated by LPS or TNF-α, act via prostaglandin E2-dependent reprogramming of host macrophages to increase their IL-10 production. Gonzalez-Rey et al. demonstrated that human adult stem cells derived from the adipose tissue are able to protect mice with dextran sulphate sodium-induced colitis from both intestinal damage and sepsis [37].
3.3 Stem Cells as a Treatment for NEC
NEC is characterized by the combination of intestinal and systemic inflammation, which in severe cases lead, respectively, to intestinal necrosis and generalized sepsis. The results obtained with mesenchymal stem cells in experimental colitis and in animal models of sepsis, together with the limited therapeutic options currently available, prompt the evaluation of the therapeutic potential of stem cells also in NEC.
To date, two groups investigated the efficacy of bone marrow-derived mesenchymal stem cells in a neonatal rat model of NEC. Notwithstanding a reduction in the severity of intestinal damage, in both studies adult bone marrow-derived mesenchymal stem cells failed to provide any significant benefit on animal survival [38,39]. The different therapeutic effect of adult mesenchymal stem cells obtained in experimental NEC vs. animal models of inflammatory bowel disease (IBD) could be linked to pathogenetic differences. In NEC, the primary causes of enterocolitis are bowel immaturity, bacterial infection and ischemia [2,3], whereas in IBDs, the pathological changes are primarily related to a dysregulation of the immune system [40]. Similarly the NEC model, comprises several pathogenic factors (i.e. ischemia, feeding with hyperosmolar formula, administration of LPS) that are directly implicated in the human disease [13], while models of IBD are usually obtained administering either dextran sodium sulphate [41] or peptidoglycan-polysaccharide [42] to animals that, in the vast majority of cases, carry genetic alterations of the immune system (e.g. IL10−/− mice) [43].
The lack of effect of adult bone marrow-derived mesenchymal stem cells in experimental NEC prompted the evaluation of a population of perinatal stem cells with higher regenerative potential and greater potency: amniotic fluid stem (AFS) cells.
AFS cells represent a unique population of stem cells of foetal origin with intermediate characteristics between embryonic stem cells and adult mesenchymal stem cells; on the one hand, they express embryonic markers, harbour a great proliferative and clonogenic potential, differentiate into multiple lineages and generate embryoid bodies and, on the other hand, possess mesenchymal markers and do not form teratomas after implantation in vivo [44,45]. Since their first isolation in 2007 [46] succeeding studies have provided evidence concerning their broad therapeutic potential in animal models of disease both via direct tissue regeneration [47, 48, 49, 50] and paracrine stimulation of tissue repair [51,52, 53, 54]. Moreover, the gut homing capacity of AFS cells when directly injected into the peritoneal cavity, even in the absence of organ damage, was recently reported along with their safety [55]. The authors injected 2 × 106 AFS cells transfected to express the green fluorescent protein (GFP) into the peritoneum of healthy newborn rats born at term via vaginal delivery and followed up the animals: none of the animals died or showed adverse effects related to the procedure. AFS cell systemic diffusion and tissue integration were evaluated by immunofluorescence (i.e. detection of GFP) and molecular biology (i.e. amplification of the gfp gene). Both techniques demonstrated that AFS cells mainly distribute in organs of the abdominal compartment, preferentially localizing in the intestine, which resulted positive for GFP in more than 60 % of treated animals. This could be determined by direct diffusion of the cells from the peritoneal surface into the intestinal wall as well as by cell spreading via lymphatic drainage or local blood circulation. Contrarily, intravenously administered AFS cells home primarily to the lung, subsequently colonize kidneys, spleen and liver, but do not distribute into the gut [52].
The potential utility of AFS cells in NEC was also supported by the publication of Good et al, who demonstrated that mouse amniotic fluid injected into the gut wall attenuates the severity of intestinal damage in experimental NEC. The protective agent in amniotic fluid appears to be the epidermal growth factor (EGF), which has an inhibitory effect on TLR4 signalling through the peroxisome proliferator-activated receptor. Inhibition of the EGF receptor with cetuximab or depletion of EGF in the amniotic fluid blocks the inhibitory effects of amniotic fluid on TLR4. Similarly, the amniotic fluid does not prevent TLR4 signalling in EGF receptor-deficient mice or peroxisome proliferator-activated receptor γ-deficient enterocytes [56].
All these data encouraged the evaluation of AFS cell therapeutic role in experimental NEC.
4 Treatment of Experimental NEC with Amniotic Fluid Stem Cells
The potential of AFS cells for the treatment NEC has been recently evaluated in a neonatal rat model of disease [39]. The effects of AFS cells on animal survival and clinical status, as well as on gut damage were primarily investigated along with stem cell mechanism of action.
4.1 Experimental Design
According to the UK Home Office regulations for Animals, a neonatal rat model of disease based on gavage feeding with hyperosmolar formula, hypoxia and oral administration of LPS was employed [22,39]. After 24 h of life, NEC rats were randomized to receive, at 24 and 48 h of life and via i.p. injection, either AFS cells or placebo (i.e. phosphate buffered saline, PBS). Further groups of animals were used as controls: breastfed (BF) rats and NEC rats injected either with adult stem cells (i.e. bone marrow-derived mesenchymal stem cells, BM-MSCs) or with fully differentiated cells (i.e. myoblasts). Animals were followed up until natural death occurred to assess survival or analysed at 96 h of life to investigate AFS cell effect on the intestine as well as post-transplantation localization and trans-differentiation (Fig. 3.3).


Fig. 3.3
Experimental design. Rats were divided in two main groups: breastfed (BF) and NEC animals. The BF group consisted of rats born at term (E21–22) via vaginal delivery from time-mated pregnant Sprague-Dawley rats; pup rats were housed with their mothers, breastfed ad libitum and not subjected to any stress. The NEC group consisted of rats delivered by Caesarean section at E21, forcedly fed by gavage with artificial milk and exposed to stress (i.e. hypoxia and LPS administration) during their first 4 days of life. At 24 h of life, NEC rats were randomized to receive, at 24 and 48 h of life and via i.p. injection, either cells (2 × 106 AFS cells, BM-MSCs or myoblasts in 50 μl of phosphate buffered saline, PBS) or placebo (i.e. 50 μl of PBS). In a first set of experiments all rats were analysed at 96 h of life, while in a second set of experiments (survival studies) rats were followed up from birth until natural death occurred
AFS cells were obtained from green fluorescent protein (GFP+) transgenic Sprague-Dawley rats at E14; clonal cell lines generated by limited dilution [46] were administered to the animals. BM-MSCs were obtained from the femurs of adult Sprague-Dawley rats following standard protocols [47]. Commercially available rat skeletal muscle myoblasts were employed.
4.2 AFS Cell Effect on Animal Mortality and Morbidity
NEC rats injected with AFS cells survive significantly longer than rats injected with PBS (p < 0.0001), myoblasts (p < 0.0001), or BM-MSCs (p = 0.024). There are no differences in terms of mortality between PBS, myoblast and BM-MSC groups (p = n.s.). As expected BF rats survive significantly longer (94 % survival) than all other groups (p < 0.0001), confirming that NEC induction is a source of mortality (Fig. 3.4a). The beneficial effect of AFS cells is robust and reproducible, even when tested on a large number of animals (Fig. 3.4b). The ability of AFS cells to lengthen survival is particularly important, as intensive care support and operative treatment given to human neonates with NEC cannot be given to pup rats and this model is therefore not compatible with long-term survival. Moreover, the specificity of this effect to AFS cells is in contrast with other animal models of bowel disease, in which BM-MSCs are effective [31, 29, 32].


Fig. 3.4
AFS cell effect on animal mortality and morbidity. (a) When treated with AFS cells, NEC rats prove to survive significantly longer than NEC rats treated with BM-MSCs (p = 0.024), PBS (p < 0.0001) and myoblasts (p < 0.0001). (b) This effect of AFS cells is extremely reproducible, as cumulative results of several experiments show a consistent survival benefit of NEC rats injected with AFS cells compared with those receiving PBS alone (p < 0.0001). (c) Morbidity, evaluated using a validated clinical sickness score, also shows a significant benefit of AFS cell treatment in comparison with PBS (p < 0.001), although AFS cell-treated rats are still sick compared with BF rats (p < 0.01)
4.3 AFS Cell Effect on Intestinal Morphology and Function
The pronounced and consistent effect of AFS cells on animal morbidity and mortality is determined by decrease of intestinal damage and preservation of gut function, as proven by different indicators.
In situ bowel assessment with high-resolution μMRI shows that, while NEC rats treated with PBS have abundant ascites, thinned gut walls and dilated bowel loops, animals treated with AFS cells are indistinguishable from BF rats (Fig. 3.5a). The radiological appearance of the gut agrees with the better macroscopical and histological appearance of the intestine of AFS cell treated rats as assessed at 96 h of life. Gut appearance of AFS rats is similar to BF rats, with significantly less damage and necrosis than PBS rats (Fig. 3.5b). At histology, AFS cells reduce the incidence of NEC from 75 % (i.e. PBS rats) to 42 % (p = 0.0009). Villus sloughing, venous congestion and villus core separation are classic histological features of NEC [22]; AFS cell-treated animals have a normal intestinal architecture with decreased incidence of all of these hallmarks (Fig. 3.5c).


Fig. 3.5
AFS cell effect on intestinal morphology and function. (a) MRI of AFS cell-treated NEC rats (left column of images) and untreated rats (right column of images). Row 1 (i and ii): degree of ascites measured using T2 maps: the total number of voxels with T2 > 160 ms identified as dark red regions which indicates areas of fluid accumulation are different between the PBS (1682 ± 453) and AFS (224 ± 135, p < 0.05) groups which do not differ from the BF group (278 ± 27). Row 2 (iii and iv): bowel wall thickness using MRI images: marked structural changes are observed in the untreated rats. Row 3 (v and vi): representative axial slices demonstrate a similar pattern. Row 4 (vii and viii): magnified images of bowel loops from the respective axial slices highlight the loss of bowel wall integrity in the untreated rats. Row 5 (ix and x): representative axial slices of BF rats and magnified image of bowel loop showing normal intestinal architecture (b, c). AFS cells decrease macroscopic and microscopic intestinal damage. (b) Examples of the macroscopic gut appearance of BF rats and NEC rats injected with PBS or AFS cells. Gut damage of NEC rats injected with AFS cells (0.4 ± 0.7, n = 27) is significantly smaller than in animals injected with PBS (1.4 ± 1.1 n = 24, p < 0.001), but shows no difference with BF rats (0.1 ± 0.3; n = 12, p = n.s.). (c) NEC rats treated with AFS cells reveal significantly less histological damage (0.23 ± 0.8, n = 48, p < 0.001) in comparison with NEC rats treated with PBS (1.78 ± 0.7, n = 50); no damage is observed in BF rats (0.08 ± 0.2, n = 12). (d) Carmine red solution administration reveals that motility is decreased in NEC rats injected with PBS (p < 0.001) but it is normal in rats injected with AFS cells (p = n.s.) when compared with BF. (e) Intestinal permeability, measured as plasma lactulose/mannitol ratio: in comparison with BF rats (0.004 ± 0.002, n = 9), PBS rats have a significant increase in intestinal permeability (0.043 ± 0.004; n = 21, p < 0.001), which is restored in AFS-treated rats (0.031 ± 0.004; n = 25, p < 0.05)
AFS cells also have a protective effect on intestinal function. Intestinal motility, measured with carmine red transit, is severely decreased in NEC rats injected with PBS in comparison with BF rats, but it is preserved in the group receiving AFS cells (Fig. 3.5d). Although gut weight and length do not differ among the groups, carmine red completes gut transit in 75 % of BF rats, 19 % of PBS rats and 47 % of those receiving AFS cells. This is confirmed by organ bath studies in which only the intestine from AFS rats shows spontaneous contractions, resembling peristalsis. Similarly, while intestinal permeability (measured by plasma lactulose/mannitol ratio) is significantly higher in PBS rats compared with BF rats, AFS cell injection in NEC rats partially prevents this increase (Fig. 3.5e).
4.4 AFS Cell Post-Transplantation Localization and Differentiation
After i.p. injection AFS cells migrate into the damaged intestine (Fig. 3.6a), integrate in the tissue and differentiate into committed cells. AFS cells exhibit various degrees of distribution mainly depending on the time occurring from their i.p. injection: after 24 h, cell bundles are adherent to the mesentery (Fig. 3.6b); at 48 h, AFS cells localize into gut wall (Fig. 3.6b); at 72 h few AFS cells migrate in the mucosa where they can express markers of mesenchymal differentiation (Fig. 3.6b, c) but not of epithelial or neuronal commitment. As demonstrated by PCR amplification of the gfp gene, the intestine is always positive for GFP (i.e. 100 % of the animals injected with AFS cells), while liver is positive in 32 % of animals, kidneys in 21 %, spleen in 20 %, heart in 17 % and lungs in 15 %; no GFP signal is ever detectable either in the brain or bones/bone marrow (Fig. 3.6d, e). Thus, while AFS cells administered intravenously home primarily in the lung and do not distribute to the gut [52], and AFS cells injected i.p. into healthy newborn rats colonize the gut in less than 70 % of the animals [55], when injected i.p. into pup rats affected by NEC AFS cells localize in the gut in 100 % of animals, homing to the mesentery or to the gut wall.


Fig. 3.6
Amniotic fluid stem (AFS) post-transplantation localization and differentiation. (a) Macroscopic appearance of bundles of AFS cells injected i.p. on the mesentery and on the gut wall. (b) GFP epifluorescence from AFS cells: 24 h after injection cells are adherent to the serosa of the intestine. At 48 h and 72 h rare AFS cells are found within the villus structure in close junction to the epithelial layer. (c) Sections of rat ileum stained with anti-GFP antibodies to localize AFS cells (green) and anti-smooth muscle actin (SMA) antibodies to label smooth muscle cells (red ) (scale bar 20 μm). AFS cells co-expressing GFP and SMA are found integrated in the mucosal layer. (d, e). PCR analysis of gfp DNA in organs of animals injected with GFP + AFS cells; AFS always migrate into the intestine (d) and in 50 % also to various other abdominal and thoracic organs (e)
4.5 AFS Cell Mechanism of Action
As suggested by the above reported data, the beneficial role of AFS cells on animal outcome and intestinal damage is closely related to their presence in the gut. However, as the improvements in morbidity and mortality occur within hours after cell administration, at which time relatively small numbers of AFS cells can be found in the bowel, their direct contribution to tissue regeneration is unlikely to be the major mechanism for AFS cell beneficial effect on the intestine. This is also in accordance with the low engraftment rate of stem cells in the intestinal wall observed at 96 h and with the restricted differentiation of engrafted cells towards the epithelial, mesenchymal and neuronal lineage at that time.
The aforementioned observations suggest that AFS cells could act by releasing one or more substance(s) harbouring a trophic effect on intestinal resident progenitor cells. This paracrine mechanism of action is demonstrated by a survival study directly comparing AFS cells vs. AFS cell-conditioned medium (CM): AFS cells and AFS cell-CM equally improve rat survival in comparison to both PBS (p < 0.01) and not conditioned medium (i.e. α-MEM; p < 0.0001) (Fig. 3.7). Moreover, by hierarchical cluster analysis of intestinal cDNA arrays, the intestine of NEC rats receiving placebo (i.e. PBS or α-MEM) is clearly distinguishable from that of animals treated with AFS cells or AFS-CM (Fig. 3.8a). Unsurprisingly, the genes with the largest expression differences are mainly involved in inflammation and tissue repair (e.g. Aoc3, Itgb6), cell cycle regulation (e.g. Atf2, Dusp16, Gpx4, Mxd1) and enterocyte differentiation processes (e.g. Acsl5, Rab8a, Thra) suggesting, in accordance to other authors [57], that damage resolution in NEC is achieved via activation of multiple pathways acting on tissue inflammation, cell apoptosis and proliferation. These gene expression differences are confirmed by biochemical and immunohistochemical assays evaluating general parameters of inflammation, epithelial proliferation and apoptosis. In NEC rats, AFS cells: reduce lipid peroxidation (MDA level; Fig. 3.8b) and neutrophil infiltration (MPO activity; Fig. 3.8c); decrease apoptosis (cleaved caspase 3 immunohistochemistry; Fig. 3.8d), particularly in the crypts (positive cells in 45 % of PBS rats with NEC vs 12 % of AFS rats, p < 0.05; Fig. 3.8e); and promote enterocyte migration from crypts to villus tips (EdU staining; Fig. 3.8f, g).


Fig. 3.7
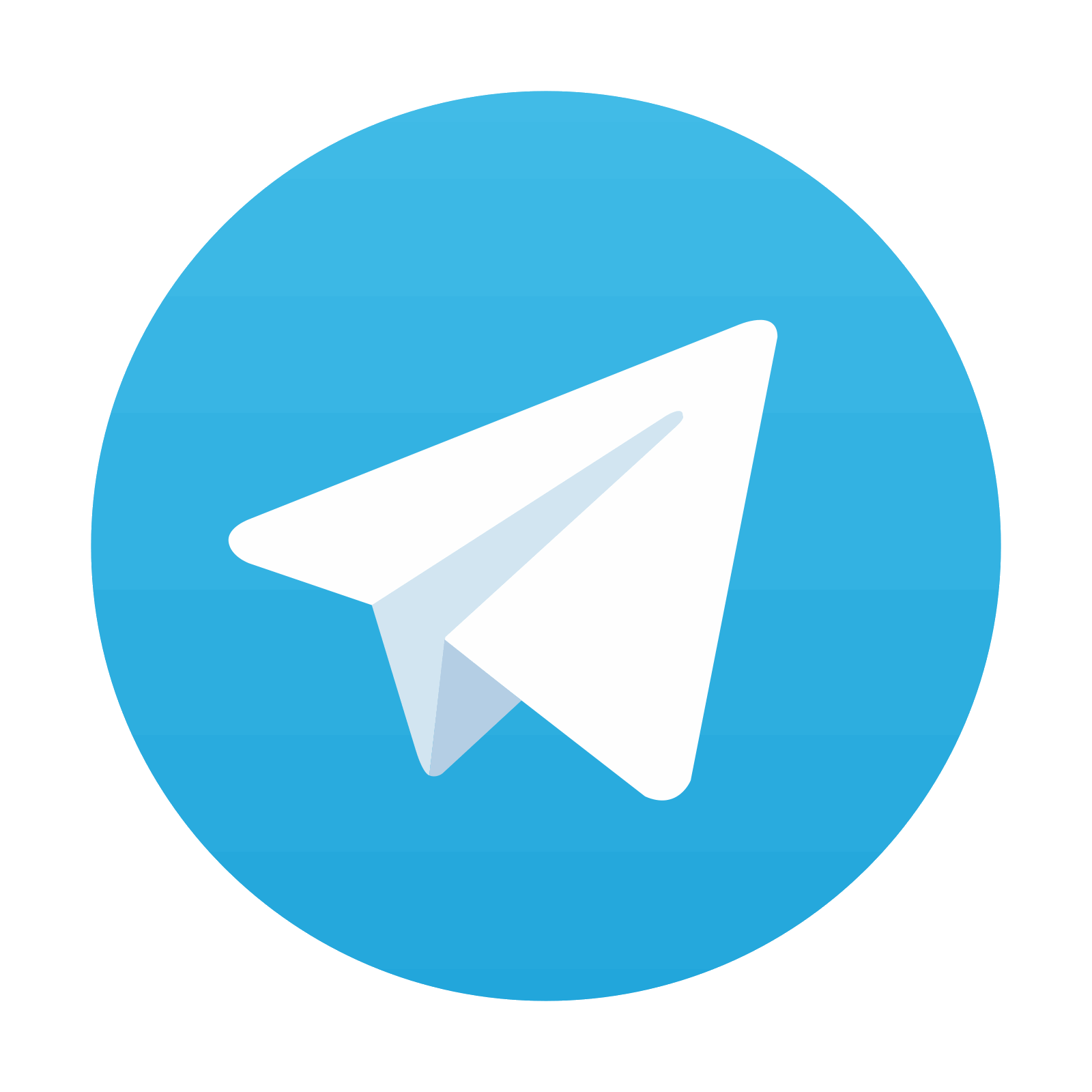
Survival study comparing the effect of AFS cells vs. AFS cell-conditioned medium. (a) Experimental design. At 24 and 48 h of life NEC rats received an i.p. injection of either: (1) 2×106 AFS cells in 50 μl of PBS (AFS cells group), (2) 50 μl of serum-free medium conditioned by AFS cells (CM group), (3) 50 μl of serum-free medium not conditioned by AFS cells (α-MEM group) and (4) 50 μl of PBS alone (PBS group). (b) The survival of rats injected with AFS cell-conditioned medium is similar to that of rats treated with AFS cells (p = n.s.), while is significantly superior to that of NEC rats injected with PBS (p < 0.01) or with α-MEM (p < 0.0001). No differences are observed among rats treated with PBS or α-MEM p = n.s.)
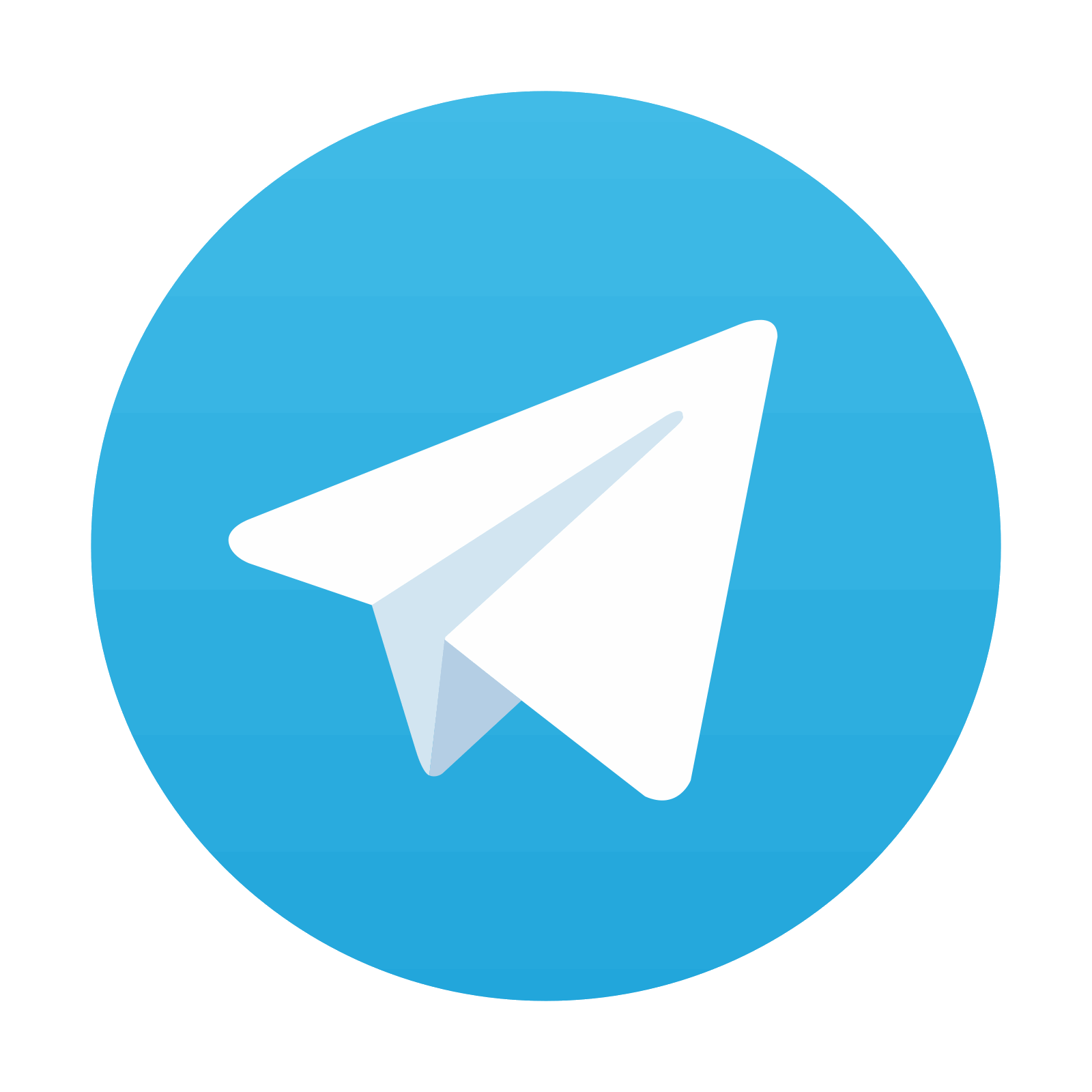
Stay updated, free articles. Join our Telegram channel
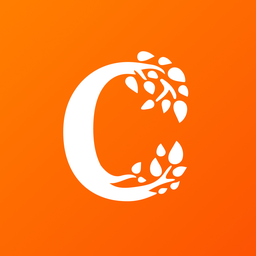
Full access? Get Clinical Tree
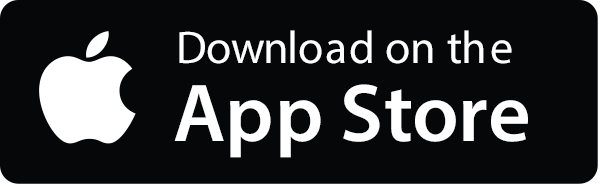
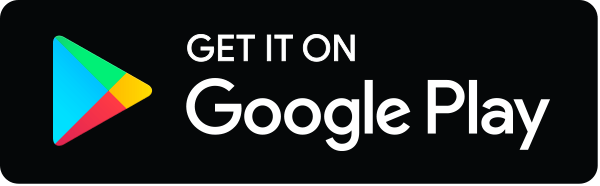
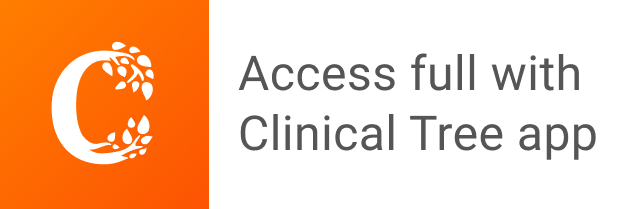