Objectives
The reader understands how oxygen and carbon dioxide are transported to and from the tissues in the blood.
- States the relationship between the partial pressure of oxygen in the blood and the amount of oxygen physically dissolved in the blood.
- Describes the chemical combination of oxygen with hemoglobin and the “oxyhemoglobin dissociation curve.”
- Defines hemoglobin saturation, the oxygen-carrying capacity, and the oxygen content of blood.
- States the physiologic consequences of the shape of the oxyhemoglobin dissociation curve.
- Lists the physiologic factors that can influence the oxyhemoglobin dissociation curve, and predicts their effects on oxygen transport by the blood.
- States the relationship between the partial pressure of carbon dioxide in the blood and the amount of carbon dioxide physically dissolved in the blood.
- Describes the transport of carbon dioxide as carbamino compounds with blood proteins.
- Explains how most of the carbon dioxide in the blood is transported as bicarbonate.
- Describes the carbon dioxide dissociation curve for whole blood.
- Explains the Bohr and Haldane effects.
Transport of Oxygen and Carbon Dioxide in the Blood: Introduction
The final step in the exchange of gases between the external environment and the tissues is the transport of oxygen and carbon dioxide to and from the lung by the blood. Oxygen is carried both physically dissolved in the blood and chemically combined to hemoglobin. Carbon dioxide is carried physically dissolved in the blood, chemically combined to blood proteins as carbamino compounds, and as bicarbonate.
Transport of Oxygen by the Blood
Oxygen is transported both physically dissolved in blood and chemically combined to the hemoglobin in the erythrocytes. Much more oxygen is normally transported combined with hemoglobin than is physically dissolved in the blood. Without hemoglobin, the cardiovascular system could not supply sufficient oxygen to meet tissue demands.
At a temperature of 37°C, 1 mL of plasma contains 0.00003 mL O2/mm Hg . This corresponds to Henry’s law, as discussed in Chapter 6. Whole blood contains a similar amount of dissolved oxygen per milliliter because oxygen dissolves in the fluid of the erythrocytes in about the same amount. Therefore, normal arterial blood with a
of approximately 100 mm Hg contains only about 0.003 mL O2/mL of blood, or 0.3 mL O2/100 mL of blood. (Blood oxygen content is conventionally expressed in milliliters of oxygen per 100 mL of blood, or volumes percent.)
A few simple calculations can demonstrate that the oxygen physically dissolved in the blood is not sufficient to fulfill the body’s oxygen demand (at normal Fio2 and barometric pressure). The resting oxygen consumption of an adult is approximately 250 to 300 mL O2/min. If the tissues were able to remove the entire 0.3 mL O2/100 mL of blood flow they receive, the cardiac output would have to be about 83.3 L/min to meet the tissue demand for oxygen at rest:
During strenuous exercise, the oxygen demand can increase as much as 16-fold to 4 L/min or more. Under such conditions, the cardiac output would have to be greater than 1000 L/min if physically dissolved oxygen were to supply all the oxygen required by the tissues. The maximum cardiac outputs attainable by normal adults during strenuous exercise are in the range of 25 L/min. Clearly, the physically dissolved oxygen in the blood cannot meet the metabolic demand for oxygen, even at rest.
Hemoglobin is a complex molecule with a molecular weight of about 64,500. The protein portion (globin) has a tetrameric structure consisting of 4 linked polypeptide chains, each of which is attached to a protoporphyrin (heme) group. Each heme group consists of 4 symmetrically arranged pyrroles with a ferrous (Fe2+) iron atom at its center. The iron atom is bound to each of the pyrrole groups and to 1 of the 4 polypeptide chains. A sixth binding site on the ferrous iron atom is freely available to bind with oxygen (or carbon monoxide). Therefore each of the 4 polypeptide chains can bind a molecule of oxygen (or carbon monoxide) to the iron atom in its own heme group, and so the tetrameric hemoglobin molecule can combine chemically with 4 oxygen molecules (or 8 oxygen atoms). Both the globin component and the heme component (with its iron atom in the ferrous state), in their proper spatial orientation to each other, are necessary for the chemical reaction with oxygen to take place—neither heme nor globin alone will combine with oxygen. Each of the tetrameric hemoglobin subunits can combine with oxygen by itself (see Figure 7–4C).
Variations in the amino acid sequences of the 4 globin subunits may have important physiologic consequences. Normal adult hemoglobin (HbA) consists of 2 alpha (α) chains, each of which has 141 amino acids, and 2 beta (β) chains, each of which has 146 amino acids. Fetal hemoglobin (HbF), which consists of 2 α chains and 2 gamma (γ) chains, has a higher affinity for oxygen than does HbA. Synthesis of β chains normally begins about 6 weeks before birth, and HbA usually replaces almost all the HbF by the time an infant is 4 months old. Other, abnormal hemoglobin molecules may be produced by genetic substitution of a single amino acid for the normal one in an α or β chain or (rarely) by alterations in the structure of heme groups. These alterations may produce changes in the affinity of the hemoglobin for oxygen, change the physical properties of hemoglobin, or alter the interaction of hemoglobin and other substances that affect its combination with oxygen, such as 2,3-bisphosphoglycerate (2,3-BPG) (discussed later in this chapter). More than 1000 abnormal variants of normal HbA have been demonstrated in patients. The best known of these, hemoglobin S, is present in sickle cell disease, an autosomal recessive genetic disorder caused by a single point mutation in the β chain. Hemoglobin S tends to polymerize and crystallize in the cytosol of the erythrocyte when it is not combined with oxygen. This polymerization and crystallization decreases the solubility of hemoglobin S within the erythrocyte and changes the shape of the cell from the normal biconcave disk to a crescent or “sickle” shape. A sickled cell is more fragile than a normal cell, causing hemolytic anemia. In addition, the cells have a tendency to stick to one another, which increases blood viscosity and also favors thrombosis or blockage of blood vessels.
Hemoglobin rapidly combines reversibly with oxygen. It is the reversibility of the reaction that allows oxygen to be released to the tissues; if the reaction did not proceed easily in both directions, hemoglobin would be of little use in delivering oxygen to satisfy metabolic needs. The reaction is very fast, with a half-time of 0.01 of a second or less. Each gram of hemoglobin is capable of combining with about 1.39 mL of oxygen under optimal conditions, but under normal circumstances some hemoglobin exists in forms such as methemoglobin (in which the iron atom is in the ferric state) or is combined with carbon monoxide, in which case the hemoglobin does not bind oxygen. For this reason, the oxygen-carrying capacity of hemoglobin is conventionally considered to be 1.34 mL O2/g Hb. That is, each gram of hemoglobin, when fully saturated with oxygen, binds 1.34 mL of oxygen. Therefore, a person with 15 g Hb/100 mL of blood has an oxygen-carrying capacity of 20.1 mL O2/100 mL of blood:
The reaction of hemoglobin and oxygen is conventionally written
Hemoglobin and the Physiologic Implications of the Oxyhemoglobin Dissociation Curve
The equilibrium point of the reversible reaction of hemoglobin and oxygen is, of course, dependent on how much oxygen the hemoglobin in blood is exposed to. This corresponds directly to the partial pressure of oxygen in the plasma under the conditions in the body. Thus, the
of the plasma determines the amount of oxygen that binds to the hemoglobin in the erythrocytes.
One way to express the proportion of hemoglobin that is bound to oxygen is as percent saturation. This is equal to the content of oxygen in the blood (minus that part physically dissolved) divided by the oxygen-carrying capacity of the hemoglobin in the blood times 100%:
Note that the oxygen-carrying capacity of an individual depends on the amount of hemoglobin in that person’s blood. The blood oxygen content also depends on the amount of hemoglobin present (as well as on the ). Both content and capacity are expressed as milliliters of oxygen per 100 mL of blood. On the other hand, the percent hemoglobin saturation expresses only a percentage and not an amount or volume of oxygen. Therefore, “percent saturation” is not interchangeable with “oxygen content.” For example, 2 patients might have the same percent of hemoglobin saturation, but if one has a lower blood hemoglobin concentration because of anemia, he or she will have a lower blood oxygen content.
The relationship between the of the plasma and the percent of hemoglobin saturation is demonstrated graphically as the oxyhemoglobin dissociation curve. An oxyhemoglobin dissociation curve for normal blood is shown in Figure 7–1.
The oxyhemoglobin dissociation curve is really a plot of how the availability of one of the reactants, oxygen (expressed as the of the plasma), affects the reversible chemical reaction of oxygen and hemoglobin. The product, oxyhemoglobin, is expressed as percent saturation—really a percentage of the maximum for any given amount of hemoglobin.
As can be seen in Figure 7–1, the relationship between and HbO2 is not linear; it is an S-shaped curve, steep at the lower
and nearly flat when the
is above 70 mm Hg.
It is this S shape that is responsible for several very important physiologic properties of the reaction of oxygen and hemoglobin. The reason that the curve is S-shaped and not linear is that it is actually a plot of 4 reactions rather than 1. That is, each of the 4 subunits of hemoglobin can combine with 1 molecule of oxygen. Indeed, it may be more correct to write the following equation:
The reactions of the 4 subunits of hemoglobin with oxygen do not appear to occur simultaneously. Instead they are believed to occur sequentially in 4 steps, with an interaction between the subunits occurring in such a way that during the successive combinations of the subunits with oxygen, each combination facilitates the next (“positive cooperativity”). Similarly, dissociation of oxygen from hemoglobin subunits facilitates further dissociations. The dissociation curve for a single monomer of hemoglobin is far different from that for the tetramer (see Figure 7–4C).
As already stated, for hemoglobin to participate in the transport of oxygen from the lungs to the tissues, it must combine with oxygen in the pulmonary capillaries and then release oxygen to the metabolizing tissues in the systemic capillaries. The oxyhemoglobin dissociation curve in Figure 7–1 shows how this is accomplished.
Mixed venous blood entering the pulmonary capillaries normally has a of about 40 mm Hg, as discussed in Chapter 5. At a
of 40 mm Hg, hemoglobin is about 75% saturated with oxygen, as seen in Figure 7–1. Assuming a blood hemoglobin concentration of 15 g Hb/100 mL of blood, this corresponds to 15.08 mL O2/100 mL of blood bound to hemoglobin plus an additional 0.12 mL O2/100 mL of blood physically dissolved, or a total oxygen content of approximately 15.2 mL O2/100 mL of blood.
Oxygen-carrying capacity is
Oxygen bound to hemoglobin at a of 40 mm Hg (37°C, pH 7.4) is
Oxygen physically dissolved at a of 40 mm Hg is
Total blood oxygen content at a of 40 mm Hg (37°C, pH 7.4) is
As the blood passes through the pulmonary capillaries, it equilibrates with the alveolar of about 100 mm Hg. At a
of 100 mm Hg, hemoglobin is about 97.4% saturated with oxygen, as seen in Figure 7–1. This corresponds to 19.58 mL O2/100 mL of blood bound to hemoglobin plus 0.3 mL O2/100 mL of blood physically dissolved, or a total oxygen content of 19.88 mL O2/100 mL of blood.
Oxygen bound to hemoglobin at a of 100 mm Hg (37°C, pH 7.4) is
Oxygen physically dissolved at a of 100 mm Hg is
Total blood oxygen content at a of 100 mm Hg (37°C, pH 7.4) is
Thus, in passing through the lungs, each 100 mL of blood has loaded (19.88 – 15.20) mL O2, or 4.68 mL O2. Assuming a cardiac output of 5 L/min, this means that approximately 234 mL O2 is loaded into the blood per minute:
Note that the oxyhemoglobin dissociation curve is relatively flat when is greater than approximately 70 mm Hg. This is very important physiologically because it means that there is only a small decrease in the oxygen content of blood equilibrated with a
of 70 mm Hg instead of 100 mm Hg. In fact, the curve shows that at a
of 70 mm Hg, hemoglobin is still approximately 94.1% saturated with oxygen. This constitutes an important safety factor because a patient with a relatively low alveolar or arterial
of 70 mm Hg (owing to hypoventilation or intrapulmonary shunting, for example) is still able to load oxygen into the blood with little difficulty. A quick calculation shows that at 70 mm Hg the total blood oxygen content is approximately 19.12 mL O2/100 mL of blood compared with the 19.88 mL O2/100 mL of blood at a
of 100 mm Hg. These calculations show that
is often a more sensitive diagnostic indicator of the status of a patient’s respiratory system than is the arterial oxygen content. Of course, the oxygen content is more important physiologically to the patient.
It should also be noted that since hemoglobin is approximately 97.4% saturated at a of 100 mm Hg, raising the alveolar
above 100 mm Hg can combine little additional oxygen with hemoglobin (only about 0.52 mL O2/100 mL of blood at a hemoglobin concentration of 15 g/100 mL of blood). Hemoglobin is fully saturated with oxygen at a
of about 250 mm Hg.
As blood passes from the arteries into the systemic capillaries, it is exposed to lower , and oxygen is released by the hemoglobin. The
in the capillaries varies from tissue to tissue, being very low in some (eg, myocardium) and relatively higher in others (eg, kidney). As can be seen in Figure 7–1, the oxyhemoglobin dissociation curve is very steep in the range of 40 to 10 mm Hg. This means that a small decrease in
can result in a substantial further dissociation of oxygen and hemoglobin, unloading more oxygen for use by the tissues. At a
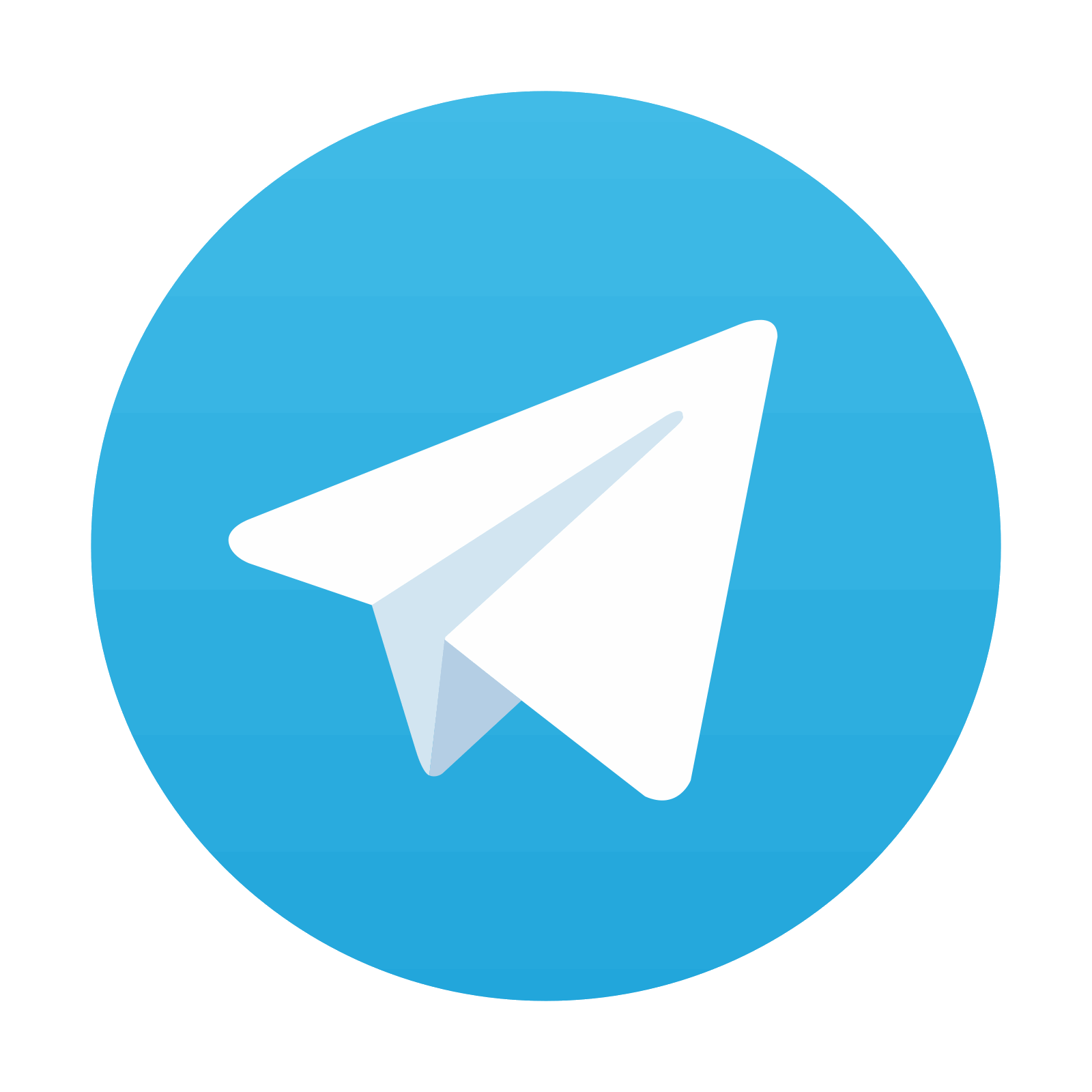
Stay updated, free articles. Join our Telegram channel
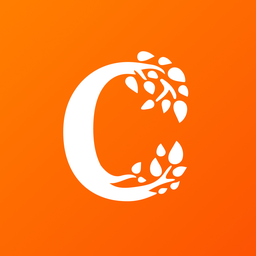
Full access? Get Clinical Tree
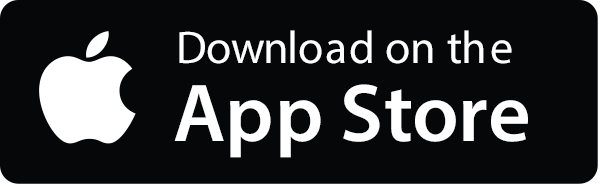
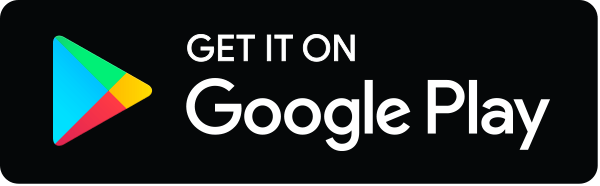
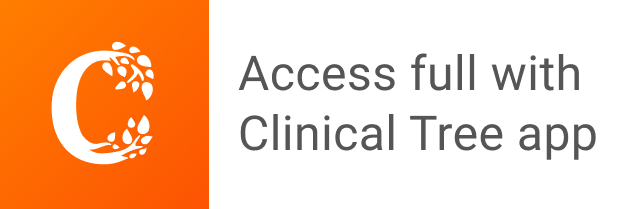