Chapter 7
The nervous system
Cells and tissues of the nervous system
The meninges and cerebrospinal fluid (CSF)
Effect of ageing on the nervous system
Infections of the central nervous system
Developmental abnormalities of the nervous system
7.1 Divisions of the nervous system 144
7.2 The neurone 145
7.3 The nerve impulse 147
7.4 The synapse 147
7.5 Brain ventricles 153
7.6 Main parts of the brain 154
7.7 Areas of the brain that control body functions 154
7.8 Vertebral column and spinal nerves showing nerve roots 161
7.9 Reflex arc of patellar impulse 164
7.10 Cranial nerves 170
7.11 Impulse conduction in the autonomic nervous system 173
7.12 Comparison of autonomic and somatic conduction system pathways 173
7.13 Autonomic nervous system neurotransmitters 173
7.14 Functions of the sympathetic nervous system 176
7.15 Functions of the parasympathetic nervous system 176
7.16 Haematomas 181
7.17 Thromboembolic stroke 182
7.18 Parkinson disease 183
The nervous system detects and responds to changes inside and outside the body. Together with the endocrine system, it coordinates and controls vital aspects of body function and maintains homeostasis. To this end the nervous system provides an immediate response while endocrine activity (Ch. 9) is, usually, slower and more prolonged.
The nervous system consists of the brain, the spinal cord and peripheral nerves (see Fig. 1.10, p. 10). The structure and organisation of the tissues that form these components enables rapid communication between all parts of the body.
For descriptive purposes the parts of the nervous system are grouped as follows:
The PNS comprises paired cranial and sacral nerves – some of these are sensory (afferent) transmitting impulses to the CNS, some are motor (efferent) transmitting impulses from the CNS and others are mixed. It is useful to consider two functional parts within the PNS:
The motor division has two parts:
• the somatic nervous system, which controls voluntary movement of skeletal muscles
• the autonomic nervous system, controlling involuntary processes such as heartbeat, peristalsis (p. 289) and glandular activity. The autonomic nervous system has two divisions: sympathetic and parasympathetic.
In summary, the CNS receives sensory information about its internal and external environments from afferent nerves. The CNS integrates and processes this input and responds, when appropriate, by sending nerve impulses through motor nerves to the effector organs: muscles and glands. For example, responses to changes in the internal environment regulate essential involuntary body functions such as respiration and blood pressure; responses to changes in the external environment maintain posture and other voluntary activities.
The first sections of this chapter explore the structure and functions of the components of the nervous system including the impact of ageing, while the final one considers the effects on body function when its structures do not function normally.
Cells and tissues of the nervous system
There are two types of nervous tissue, neurones and neuroglia. Neurones (nerve cells) are the working units of the nervous system that generate and transmit nerve impulses. Neurones are supported by connective tissue, collectively known as neuroglia, which is formed from different types of glial cells. There are vast numbers of both cell types, 1 trillion (1012) glial cells and 10 times fewer (1011) neurones.
Neurones (Fig. 7.2)
7.2
Each neurone (Fig. 7.2) consists of a cell body and its processes, one axon and many dendrites. Neurones are commonly referred to as nerve cells. Bundles of axons bound together are called nerves. Neurones cannot divide, and for survival they need a continuous supply of oxygen and glucose. Unlike many other cells, neurones can synthesise chemical energy (ATP) only from glucose.
Neurones generate and transmit electrical impulses called action potentials. The initial strength of the impulse is maintained throughout the length of the neurone. Some neurones initiate nerve impulses while others act as ‘relay stations’ where impulses are passed on and sometimes redirected.
Nerve impulses can be initiated in response to stimuli from:
Transmission of nerve signals is both electrical and chemical. The action potential travelling down the nerve axon is an electrical signal, but because nerves do not come into direct contact with each other, the signal between a nerve cell and the next cell in the chain is nearly always chemical (p. 148).
Cell bodies
Nerve cells vary considerably in size and shape but they are all too small to be seen by the naked eye. Cell bodies form the grey matter of the nervous system and are found at the periphery of the brain and in the centre of the spinal cord. Groups of cell bodies are called nuclei in the central nervous system and ganglia in the peripheral nervous system. An important exception is the basal ganglia (nuclei) situated within the cerebrum (p. 156).
Axons and dendrites
Axons and dendrites are extensions of cell bodies and form the white matter of the nervous system. Axons are found deep in the brain and in groups, called tracts, at the periphery of the spinal cord. They are referred to as nerves or nerve fibres outside the brain and spinal cord.
Axons
Each nerve cell has only one axon, which begins at a tapered area of the cell body, the axon hillock. They carry impulses away from the cell body and are usually longer than the dendrites, sometimes as long as 100 cm.
Structure of an axon.
The membrane of the axon is called the axolemma and it encloses the cytoplasmic extension of the cell body.
Myelinated neurones
Large axons and those of peripheral nerves are surrounded by a myelin sheath (Figs 7.3A and C). This consists of a series of Schwann cells arranged along the length of the axon. Each one is wrapped around the axon so that it is covered by a number of concentric layers of Schwann cell plasma membrane. Between the layers of plasma membrane is a small amount of fatty substance called myelin. The outermost layer of the Schwann cell plasma membrane is the neurilemma. There are tiny areas of exposed axolemma between adjacent Schwann cells, called nodes of Ranvier (Fig. 7.2), which assist the rapid transmission of nerve impulses in myelinated neurones. Figure 7.4 shows a section through a nerve fibre at a node of Ranvier where the area without myelin can be clearly seen.
Figure 7.3 Arrangement of myelin. A. Myelinated neurone. B. Unmyelinated neurone. C. Length of myelinated axon.
Unmyelinated neurones
Postganglionic fibres and some small fibres in the central nervous system are unmyelinated. In this type a number of axons are embedded in one Schwann cell (Fig. 7.3B). The adjacent Schwann cells are in close association and there is no exposed axolemma. The speed of transmission of nerve impulses is significantly slower in unmyelinated fibres.
Dendrites
These are the many short processes that receive and carry incoming impulses towards cell bodies. They have the same structure as axons but are usually shorter and branching. In motor neurones dendrites form part of synapses (see Fig. 7.7) and in sensory neurones they form the sensory receptors that respond to specific stimuli.
The nerve impulse (action potential)
7.3
An impulse is initiated by stimulation of sensory nerve endings or by the passage of an impulse from another nerve. Transmission of the impulse, or action potential, is due to movement of ions across the nerve cell membrane. In the resting state the nerve cell membrane is polarised due to differences in the concentrations of ions across the plasma membrane. This means that there is a different electrical charge on each side of the membrane, which is called the resting membrane potential. At rest the charge on the outside is positive and inside it is negative. The principal ions involved are:
In the resting state there is a continual tendency for these ions to diffuse along their concentration gradients, i.e. K+ outwards and Na+ into cells. When stimulated, the permeability of the nerve cell membrane to these ions changes. Initially Na+ floods into the neurone from the extracellular fluid causing depolarisation, creating a nerve impulse or action potential. Depolarisation is very rapid, enabling the conduction of a nerve impulse along the entire length of a neurone in a few milliseconds. It passes from the point of stimulation in one direction only, i.e. away from the point of stimulation towards the area of resting potential. The one-way direction of transmission is ensured because following depolarisation it takes time for repolarisation to occur.
Almost immediately following the entry of Na+, K+ floods out of the neurone and the movement of these ions returns the membrane potential to its resting state. This is called the refractory period during which restimulation is not possible. The action of the sodium–potassium pump expels Na+ from the cell in exchange for K+ (see p. 37) returning levels of Na+ and K+ to the original resting state, repolarizing the neurone.
In myelinated neurones, the insulating properties of the myelin sheath prevent the movement of ions. Therefore electrical changes across the membrane can only occur at the gaps in the myelin sheath, i.e. at the nodes of Ranvier (see Fig. 7.2). When an impulse occurs at one node, depolarisation passes along the myelin sheath to the next node so that the flow of current appears to ‘leap’ from one node to the next. This is called saltatory conduction (Fig. 7.5).
The speed of conduction depends on the diameter of the neurone: the larger the diameter, the faster the conduction. In addition, myelinated fibres conduct impulses faster than unmyelinated fibres because saltatory conduction is faster than continuous conduction, or simple propagation (Fig. 7.6). The fastest fibres can conduct impulses to, e.g., skeletal muscles at a rate of 130 metres per second while the slowest impulses travel at 0.5 metres per second.
The synapse and neurotransmitters
7.4
There is always more than one neurone involved in the transmission of a nerve impulse from its origin to its destination, whether it is sensory or motor. There is no physical contact between two neurones. The point at which the nerve impulse passes from the presynaptic neurone to the postsynaptic neurone is the synapse (Fig. 7.7). At its free end, the axon of the presynaptic neurone breaks up into minute branches that terminate in small swellings called synaptic knobs, or terminal boutons. These are in close proximity to the dendrites and the cell body of the postsynaptic neurone. The space between them is the synaptic cleft. Synaptic knobs contain spherical membrane bound synaptic vesicles, which store a chemical, the neurotransmitter that is released into the synaptic cleft. Neurotransmitters are synthesised by nerve cell bodies, actively transported along the axons and stored in the synaptic vesicles. They are released by exocytosis in response to the action potential and diffuse across the synaptic cleft. They act on specific receptor sites on the postsynaptic membrane. Their action is short lived, because immediately they have acted on the postsynaptic cell such as a muscle fibre, they are either inactivated by enzymes or taken back into the synaptic knob. Some important drugs mimic, neutralise (antagonise) or prolong neurotransmitter activity. Neurotransmitters usually have an excitatory effect on postsynaptic receptors but they are sometimes inhibitory.
There are more than 50 neurotransmitters in the brain and spinal cord including noradrenaline (norepinephrine), adrenaline (epinephrine), dopamine, histamine, serotonin, gamma aminobutyric acid (GABA) and acetylcholine. Other substances, such as enkephalins, endorphins and substance P, have specialised roles in, for example, transmission of pain signals. Figure 7.8 summarises the main neurotransmitters of the peripheral nervous system.
Somatic nerves carry impulses directly to the synapses at skeletal muscles, the neuromuscular junctions (p. 422) stimulating contraction. In the autonomic nervous system (see p. 173), efferent impulses travel along two neurones (preganglionic and postganglionic) and across two synapses to the effector tissue, i.e. cardiac muscle, smooth muscle and glands, in both the sympathetic and the parasympathetic divisions.
Nerves
A nerve consists of numerous neurones collected into bundles (bundles of nerve fibres in the central nervous system are known as tracts). For example large nerves such as the sciatic nerves (p. 169) contain tens of thousands of axons. Each bundle has several coverings of protective connective tissue (Fig. 7.9):
Figure 7.9 Transverse section of a peripheral nerve showing the protective connective tissue coverings.
Sensory or afferent nerves
Sensory nerves carry information from the body to the spinal cord (Fig. 7.1). The impulses may then pass to the brain or to connector neurones of reflex arcs in the spinal cord (see p. 164).
Sensory receptors
Specialised endings of sensory neurones respond to different stimuli (changes) inside and outside the body.
Somatic, cutaneous or common senses.
These originate from the skin. They are: pain, touch, heat and cold. Sensory nerve endings in the skin are fine branching filaments without myelin sheaths (see Fig. 14.4, p. 364). When stimulated, an impulse is generated and transmitted by the sensory nerves to the brain where the sensation is perceived.
Proprioceptor senses.
These originate in muscles and joints. Impulses sent to the brain enable perception of the position of the body and its parts in space maintaining posture and balance (see Ch. 16).
Autonomic afferent nerves.
These originate in internal organs, glands and tissues, e.g. baroreceptors involved in the control of blood pressure (Ch. 5), chemoreceptors involved in the control of respiration (Ch. 10), and are associated with reflex regulation of involuntary activity and visceral pain.
Motor or efferent nerves
Motor nerves originate in the brain, spinal cord and autonomic ganglia. They transmit impulses to the effector organs: muscles and glands (Fig. 7.1). There are two types:
Mixed nerves
In the spinal cord, sensory and motor nerves are arranged in separate groups, or tracts. Outside the spinal cord, when sensory and motor nerves are enclosed within the same sheath of connective tissue they are called mixed nerves.
Neuroglia
The neurones of the central nervous system are supported by non-excitable glial cells that greatly outnumber the neurones (Fig. 7.10). Unlike nerve cells, which cannot divide, glial cells continue to replicate throughout life. There are four types: astrocytes, oligodendrocytes, ependymal cells and microglia.
Figure 7.10 Neurones and glial cells. A stained light micrograph of neurones (gold) and nuclei of the more numerous glial cells (blue).
Astrocytes
These cells form the main supporting tissue of the central nervous system (Fig. 7.11). They are star shaped with fine branching processes and they lie in a mucopolysaccharide ground substance. At the free ends of some of the processes are small swellings called foot processes. Astrocytes are found in large numbers adjacent to blood vessels with their foot processes forming a sleeve round them. This means that the blood is separated from the neurones by the capillary wall and a layer of astrocyte foot processes which together constitute the blood–brain barrier (Fig. 7.12).
Oligodendrocytes
These cells are smaller than astrocytes and are found in clusters round nerve cell bodies in grey matter, where they are thought to have a supportive function. They are found adjacent to, and along the length of, myelinated nerve fibres. Oligodendrocytes form and maintain myelin like Schwann cells in peripheral nerves.
Ependymal cells
These cells form the epithelial lining of the ventricles of the brain and the central canal of the spinal cord. Those cells that form the choroid plexuses of the ventricles secrete cerebrospinal fluid.
Microglia
The smallest and least numerous glial cells, these cells may be derived from monocytes that migrate from the blood into the nervous system before birth. They are found mainly in the area of blood vessels. They enlarge and become phagocytic, removing microbes and damaged tissue, in areas of inflammation and cell destruction.
Response of nervous tissue to injury
Neurones reach maturity a few weeks after birth and cannot be replaced.
Damage to neurones can either lead to rapid necrosis with sudden acute functional failure, or to slow atrophy with gradually increasing dysfunction. These changes are associated with:
Peripheral nerve regeneration (Fig. 7.13)
The axons of peripheral nerves can sometimes regenerate if the cell body remains intact. Distal to the damage, the axon and myelin sheath disintegrate and are removed by macrophages; the muscle supplied by the damaged fibre atrophies in the absence of nerve stimulation. The neurilemma then regenerates (about 1.5 mm per day) from the point of injury towards the effector along its original track provided the two parts of the neurilemma are in close apposition (Fig. 7.13A). New Schwann cells develop within the neurilemma providing a pathway within which the axon can regenerate.
Restoration of function depends on the re-establishment of satisfactory neural connections with the effector organ. When the neurilemma is out of position or destroyed, the sprouting axons and Schwann cells form a tumour-like cluster (traumatic neuroma) producing severe pain, e.g. following some fractures and amputation of limbs (Fig. 7.13B).
Neuroglial damage
Astrocytes.
When these cells are damaged, their processes multiply forming a mesh or ‘scar’, which is thought to inhibit the regrowth of damaged CNS neurones.
These cells increase in number around degenerating neurones and are destroyed in demyelinating diseases such as multiple sclerosis (p. 185).
Microglia.
Where there is inflammation and cell destruction the microglia increase in size and become phagocytic.
Central nervous system
The central nervous system consists of the brain and the spinal cord (see Fig. 7.1). These essential structures are both well protected from damage and injury; the brain is enclosed within the skull and the spinal cord by the vertebrae that form the spinal column. Membranous coverings known as the meninges provide further protection. The structure and functions of the meninges, brain and spinal cord are explored in this section.
The meninges and cerebrospinal fluid (CSF)
The meninges (Fig. 7.14)
The brain and spinal cord are completely surrounded by three layers of tissue, the meninges, lying between the skull and the brain, and between the vertebral foramina and the spinal cord. Named from outside inwards they are the:
The dura and arachnoid maters are separated by a potential space, the subdural space. The arachnoid and pia maters are separated by the subarachnoid space, containing cerebrospinal fluid.
Dura mater
The cerebral dura mater consists of two layers of dense fibrous tissue. The outer layer takes the place of the periosteum on the inner surface of the skull bones and the inner layer provides a protective covering for the brain. There is only a potential space between the two layers except where the inner layer sweeps inwards between the cerebral hemispheres to form the falx cerebri; between the cerebellar hemispheres to form the falx cerebelli; and between the cerebrum and cerebellum to form the tentorium cerebelli.
Venous blood from the brain drains into venous sinuses between the two layers of dura mater. The superior sagittal sinus is formed by the falx cerebri, and the tentorium cerebelli forms the straight and transverse sinuses (see Figs 5.34 and 5.35, p. 106).
Spinal dura mater forms a loose sheath round the spinal cord, extending from the foramen magnum to the 2nd sacral vertebra. Thereafter it encloses the filum terminale and fuses with the periosteum of the coccyx. It is an extension of the inner layer of cerebral dura mater and is separated from the periosteum of the vertebrae and ligaments within the neural canal by the epidural space (see Fig. 7.26), containing blood vessels and areolar connective tissue. It is attached to the foramen magnum and by strands of fibrous tissue to the posterior longitudinal ligament at intervals along its length. Nerves entering and leaving the spinal cord pass through the epidural space. These attachments stabilise the spinal cord in the neural canal. Dyes, used for diagnostic purposes, and local anaesthetics or analgesics to relieve pain, may be injected into the epidural space.
Arachnoid mater
This is a layer of fibrous tissue that lies between the dura and pia maters. It is separated from the dura mater by the subdural space that contains a small amount of serous fluid, and from the pia mater by the subarachnoid space, which contains cerebrospinal fluid. The arachnoid mater passes over the convolutions of the brain and accompanies the inner layer of dura mater in the formation of the falx cerebri, tentorium cerebelli and falx cerebelli. It continues downwards to envelop the spinal cord and ends by merging with the dura mater at the level of the 2nd sacral vertebra.
Pia mater
This is a delicate layer of connective tissue containing many minute blood vessels. It adheres to the brain, completely covering the convolutions and dipping into each fissure. It continues downwards surrounding the spinal cord. Beyond the end of the cord it continues as the filum terminale, pierces the arachnoid tube and goes on, with the dura mater, to fuse with the periosteum of the coccyx.
Ventricles of the brain and the cerebrospinal fluid
7.5
The brain contains four irregular-shaped cavities, or ventricles, containing cerebrospinal fluid (CSF) (Fig. 7.15). They are:
Figure 7.15 The positions of the ventricles of the brain (in blue) superimposed on its surface. Viewed from the left side.
The lateral ventricles
These cavities lie within the cerebral hemispheres, one on each side of the median plane just below the corpus callosum. They are separated from each other by a thin membrane, the septum lucidum, and are lined with ciliated epithelium. They communicate with the third ventricle by interventricular foramina.
The third ventricle
The third ventricle is a cavity situated below the lateral ventricles between the two parts of the thalamus. It communicates with the fourth ventricle by a canal, the cerebral aqueduct.
The fourth ventricle
The fourth ventricle is a diamond-shaped cavity situated below and behind the third ventricle, between the cerebellum and pons. It is continuous below with the central canal of the spinal cord and communicates with the subarachnoid space by foramina in its roof. Cerebrospinal fluid enters the subarachnoid space through these openings and through the open distal end of the central canal of the spinal cord.
Cerebrospinal fluid (CSF)
Cerebrospinal fluid is secreted into each ventricle of the brain by choroid plexuses. These are vascular areas where there is a proliferation of blood vessels surrounded by ependymal cells in the lining of ventricle walls. CSF passes back into the blood through tiny diverticula of arachnoid mater, called arachnoid villi (arachnoid granulations, Fig. 7.16), which project into the venous sinuses. The movement of CSF from the subarachnoid space to venous sinuses depends upon the difference in pressure on each side of the walls of the arachnoid villi, which act as one-way valves. When CSF pressure is higher than venous pressure, CSF is pushed into the blood and when the venous pressure is higher the arachnoid villi collapse, preventing the passage of blood constituents into the CSF. There may also be some reabsorption of CSF by cells in the walls of the ventricles.
From the roof of the fourth ventricle CSF flows through foramina into the subarachnoid space and completely surrounds the brain and spinal cord (Fig. 7.16). There is no intrinsic system of CSF circulation but its movement is aided by pulsating blood vessels, respiration and changes of posture.
CSF is secreted continuously at a rate of about 0.5 mL per minute, i.e. 720 mL per day. The volume remains fairly constant at about 150 mL, as absorption keeps pace with secretion. CSF pressure may be measured using a vertical tube attached to a lumbar puncture needle inserted into the subarachnoid space above or below the 4th lumbar vertebra (which is below the end of the spinal cord). The pressure remains fairly constant at about 10 cm H2O when lying on one side and about 30 cm H2O when sitting up. If the brain is enlarged by, e.g., haemorrhage or tumour, some compensation is made by a reduction in the amount of CSF. When the volume of brain tissue is reduced, such as in degeneration or atrophy, the volume of CSF is increased. CSF is a clear, slightly alkaline fluid with a specific gravity of 1.005, consisting of:
Functions of cerebrospinal fluid
CSF supports and protects the brain and spinal cord by maintaining a uniform pressure around these vital structures and acting as a cushion or shock absorber between the brain and the skull.
It keeps the brain and spinal cord moist and there may be exchange of nutrients and waste products between CSF and the interstitial fluid of the brain. CSF is thought to be involved in regulation of breathing as it bathes the surface of the medulla where the central respiratory chemoreceptors are located (Ch. 10).
Brain
The brain is a large organ weighing around 1.4 kg that lies within the cranial cavity. Its parts are (Fig. 7.17):
Blood supply and venous drainage
The circulus arteriosus and its contributing arteries (see Fig. 5.31, p. 105) play a vital role in maintaining a constant supply of oxygen and glucose to the brain when the head is moved and also if a contributing artery is narrowed. The brain receives about 15% of the cardiac output, approximately 750 mL of blood per minute. Autoregulation keeps blood flow to the brain constant by adjusting the diameter of the arterioles across a wide range of arterial blood pressure (about 65–140 mmHg) with changes occurring only outside these limits.
Venous blood from the brain drains into the dural venous sinuses and then downwards into the internal jugular veins (see Figs 5.34 and 5.35, p. 106).
Cerebrum
This is the largest part of the brain and it occupies the anterior and middle cranial fossae (see Fig. 16.11, p. 398). It is divided by a deep cleft, the longitudinal cerebral fissure, into right and left cerebral hemispheres, each containing one of the lateral ventricles. Deep within the brain, the hemispheres are connected by a mass of white matter (nerve fibres) called the corpus callosum. The falx cerebri is formed by the dura mater (see Fig. 7.14). It separates the two cerebral hemispheres and penetrates to the depth of the corpus callosum. The superficial part of the cerebrum is composed of nerve cell bodies (grey matter), forming the cerebral cortex, and the deeper layers consist of nerve fibres (axons, white matter).
The cerebral cortex shows many infoldings or furrows of varying depth. The exposed areas of the folds are the gyri (convolutions) and these are separated by sulci (fissures). These convolutions greatly increase the surface area of the cerebrum.
For descriptive purposes each hemisphere of the cerebrum is divided into lobes which take the names of the bones of the cranium under which they lie:
The boundaries of the lobes are marked by deep sulci. These are the central, lateral and parieto-occipital sulci (Fig. 7.18).
Cerebral tracts and basal ganglia (Fig. 7.19)
The surface of the cerebral cortex is composed of grey matter (nerve cell bodies). Within the cerebrum the lobes are connected by masses of nerve fibres, or tracts, which make up the white matter of the brain. The afferent and efferent fibres linking the different parts of the brain and spinal cord are as follows.
The internal capsule (Fig. 7.19) is an important projection tract that lies deep within the brain between the basal ganglia and the thalamus. Many nerve impulses passing to and from the cerebral cortex are carried by fibres that form the internal capsule. Motor fibres within the internal capsule form the pyramidal tracts (corticospinal tracts) that cross over (decussate) at the medulla oblongata and are the main pathway to skeletal muscles. Those motor fibres that do not pass through the internal capsule form the extrapyramidal tracts and have connections with many parts of the brain including the basal ganglia, thalamus and cerebellum.
Basal ganglia
The basal ganglia are groups of cell bodies that lie deep within the brain and form part of the extrapyramidal tracts. They act as relay stations with connections to many parts of the brain including motor areas of the cerebral cortex and thalamus. Their functions include initiation and fine control of complex movement and learned coordinated activities, such as posture and walking. If control is inadequate or absent, movements are jerky, clumsy and uncoordinated.
Functions of the cerebral cortex
There are three main types of activity associated with the cerebral cortex:
Functional areas of the cerebral cortex (Fig. 7.20)
The main functional areas of the cerebral cortex have been identified but it is unlikely that any area is associated exclusively with only one function. Except where specially mentioned, the different areas are active in both hemispheres; however, there is some variation between individuals. There are different types of functional area:
• motor, which direct skeletal (voluntary) muscle movements
• sensory, which receive and decode sensory impulses enabling sensory perception
In general, areas of the cortex lying anterior to the central sulcus are associated with motor functions, and those lying posterior to it are associated with sensory functions.
Motor areas of the cerebral cortex
The primary motor area.
This lies in the frontal lobe immediately anterior to the central sulcus. The cell bodies are pyramid shaped (Betz’s cells) and they control skeletal muscle activity. Two neurones involved in the pathway to skeletal muscle. The first, the upper motor neurone, descends from the motor cortex through the internal capsule to the medulla oblongata. Here it crosses to the opposite side and descends in the spinal cord. At the appropriate level in the spinal cord it synapses with a second neurone (the lower motor neurone), which leaves the spinal cord and travels to the target muscle. It terminates at the motor end plate of a muscle fibre (Fig. 7.21). This means that the motor area of the right hemisphere of the cerebrum controls voluntary muscle movement on the left side of the body and vice versa. Damage to either of these neurones may result in paralysis.
In the motor area of the cerebrum the body is represented upside down, i.e. the uppermost cells control the feet and those in the lowest part control the head, neck, face and fingers (Fig. 7.22A). The sizes of the areas of cortex representing different parts of the body are proportional to the complexity of movement of the body part, not to its size. Figure 7.22A shows that – in comparison with the trunk – the hand, foot, tongue and lips are represented by large cortical areas reflecting the greater degree of motor control associated with these areas.
Motor speech (Broca’s) area.
This is situated in the frontal lobe just above the lateral sulcus and controls the muscle movements needed for speech. It is dominant in the left hemisphere in right-handed people and vice versa.
Sensory areas of the cerebral cortex
The somatosensory area.
This is the area immediately behind the central sulcus. Here sensations of pain, temperature, pressure and touch, awareness of muscular movement and the position of joints (proprioception) are perceived. The somatosensory area of the right hemisphere receives impulses from the left side of the body and vice versa. The size of the cortical areas representing different parts of the body (Fig. 7.22B) is proportional to the extent of sensory innervation, e.g. the large area for the face is consistent with the extensive sensory nerve supply by the three branches of the trigeminal nerves (5th cranial nerves).
The auditory (hearing) area.
This lies immediately below the lateral sulcus within the temporal lobe. The nerve cells receive and interpret impulses transmitted from the inner ear by the cochlear (auditory) part of the vestibulocochlear nerves (8th cranial nerves).
The olfactory (smell) area.
This lies deep within the temporal lobe where impulses from the nose, transmitted via the olfactory nerves (1st cranial nerves), are received and interpreted.
The taste area.
This lies just above the lateral sulcus in the deep layers of the somatosensory area. Here, impulses from sensory receptors in taste buds are received and perceived as taste.
This lies behind the parieto-occipital sulcus and includes the greater part of the occipital lobe. The optic nerves (2nd cranial nerves) pass from the eye to this area, which receives and interprets the impulses as visual impressions.
Association areas
These are connected to each other and other areas of the cerebral cortex by association tracts and some are outlined below. They receive, coordinate and interpret impulses from the sensory and motor cortices permitting higher cognitive abilities and, although Figure 7.23 depicts some of the areas involved, their functions are much more complex.
Figure 7.23 Areas of the cerebral cortex involved in higher mental functions. A. Motor speech (Broca’s) area. B. Sensory speech (Wernicke’s) area. C. Parieto-occipital area.
The premotor area.
This lies in the frontal lobe immediately anterior to the motor area. The neurones here coordinate movement initiated by the primary motor cortex, ensuring that learned patterns of movement can be repeated. For example, in tying a shoelace or writing, many muscles contract but the movements must be coordinated and carried out in a particular sequence. Such a pattern of movement, when established, is described as manual dexterity.
The prefrontal area.
This extends anteriorly from the premotor area to include the remainder of the frontal lobe. It is a large area and is more highly developed in humans than in other animals. Intellectual functions controlled here include perception and comprehension of the passage of time, the ability to anticipate consequences of events and the normal management of emotions.
Sensory speech (Wernicke’s) area.
This is situated in the temporal lobe adjacent to the parieto-occipitotemporal area. It is here that the spoken word is perceived, and comprehension and intelligence are based. Understanding language is central to higher mental functions as they are language based. This area is dominant in the left hemisphere in right-handed people and vice versa.
The parieto-occipitotemporal area
This lies behind the somatosensory area and includes most of the parietal lobe. Its functions are thought to include spatial awareness, interpreting written language and the ability to name objects (Fig. 7.23). It has been suggested that objects can be recognised by touch alone because of the knowledge from past experience (memory) retained in this area.
Diencephalon (see Fig. 7.17)
This connects the cerebrum and the midbrain. It consists of several structures situated around the third ventricle, the main ones being the thalamus and hypothalamus, which are considered here. The pineal gland (p. 228) and the optic chiasma (p. 199) are situated there.
Thalamus
This consists of two masses of grey and white matter situated within the cerebral hemispheres just below the corpus callosum, one on each side of the third ventricle (Fig. 7.19). Sensory receptors in the skin and viscera send information about touch, pain and temperature, and input from the special sense organs travels to the thalamus where there is recognition, although only in a basic form, as refined perception also involves other parts of the brain. It is thought to be involved in the processing of some emotions and complex reflexes. The thalamus relays and redistributes impulses from most parts of the brain to the cerebral cortex.
Hypothalamus
The hypothalamus is a small but important structure which weighs around 7 g and consists of a number of nuclei. It is situated below and in front of the thalamus, immediately above the pituitary gland. The hypothalamus is linked to the posterior lobe of the pituitary gland by nerve fibres and to the anterior lobe by a complex system of blood vessels. Through these connections, the hypothalamus controls the output of hormones from both lobes of the pituitary gland (see p. 217).
Other functions of the hypothalamus include control of:
• the autonomic nervous system (p. 173)
• emotional reactions, e.g. pleasure, fear, rage
• sexual behaviour and child rearing
Brain stem (Fig. 7.17)
Midbrain
The midbrain is the area of the brain situated around the cerebral aqueduct (see Fig. 7.15) between the cerebrum above and the pons below. It consists of nuclei and nerve fibres (tracts), which connect the cerebrum with lower parts of the brain and with the spinal cord. The nuclei act as relay stations for the ascending and descending nerve fibres and have important roles in auditory and visual reflexes.
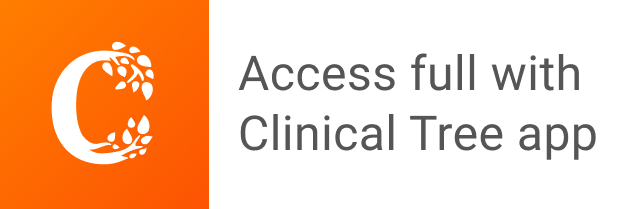