Fig. 5.1
Conjugate planes in the optical microscope (cutaway). In this cutaway of a modern microscope, “image” planes are marked in black while “aperture” planes are marked in red, denoting the two conjugate sets of light planes (Figure used by permission of Michael W. Davidson of the National High Magnetic Field Laboratory and MicroscopyU.com)
Köhler Illumination
Proper centering and focusing of the condenser is necessary for the full and even illumination that is essential for good resolution and high-quality imaging. Köhler illumination, developed in the late nineteenth century by August Köhler, completely defocuses the inherently uneven light source (such as an incandescent filament) in the image plane resulting in a very even field of illumination, while focusing the light source in the aperture plane for optimal brightness and resolution. Setting Köhler illumination requires a lamp with a collector lens to focus light at the front aperture of a focusable and centerable condenser. A simple method for setting basic Köhler illumination follows:
1.
Using a low-magnification objective, place a specimen on the stage and bring it into focus. Remove the specimen.
2.
Close down the field aperture, typically located at the base of the microscope, so that the shutter blades are visible in the field of view.
3.
Rack the condenser up or down until the field aperture blades come into sharp focus and center it in the field of view.
4.
Reopen the field diaphragm to the field of view, whether eye or digital detector.
This aligns the illuminating components into precise physical locations resulting in optimal illumination. Köhler illumination is also the starting point for the proper operation of various advanced contrasting techniques and should be performed each time the microscope is used and for individual objectives. Figure 5.2 shows the conjugate planes of a microscope aligned for Köhler illumination in both the illuminating and image-forming light paths.


Fig. 5.2
Conjugate planes in the optical microscope (light paths). The ray-trace shown in red illustrates the path taken by light focused at or originating from an aperture plane. The ray-trace shown in yellow illustrates the path taken by light focused at or originating from an image plane (Figure used by permission of Michael W. Davidson of the National High Magnetic Field Laboratory and MicroscopyU.com)
Transmitted Light Source
The transmitted light source for brightfield microscopy is usually located in an external housing or the microscope base and is most commonly an incandescent tungsten-halogen bulb. Some newer microscopes have integrated light-emitting diodes (LEDs). The housing reflects as much light as possible toward the collector lens, which then directs light into the microscope condenser. In some cases, the light source is manually centered and focused, but most modern housings automatically center the bulb. Halogen bulbs produce heat and often require some amount of ventilation, while LEDs operate at much cooler temperatures and have extended life.
An adjustable rheostat, located on the microscope body or the external power supply, regulates the voltage delivered to the bulb and adjusts the intensity of the light. With incandescent bulbs, such voltage adjustment also changes the color temperature, and this can cause major changes to the hue or color property of the image, particularly when combined with digital imaging. Neutral density filters (NDs) can be used to control the light intensity without changing the color balance by attenuating light evenly across the entire spectrum. While digital cameras have greater ability than film to adjust for changes in color temperature by automatically “white balancing,” which digitally shifts the hue of colors in relation to each other, the use of NDs to adjust light intensity reduces this need. Unlike incandescent bulbs, LEDs have a constant color temperature regardless of voltage adjustment.
Halogen bulbs emit a continuous spectrum of light that extends from about 300 to 1,400 nm. The collector lens for the lamp typically blocks ultraviolet (UV) light, while a separate infrared (IR) filter may be used to block IR light that can cause eye strain and high background on digital images. LEDs tuned for white light do not emit UV or IR light, thus reducing eye strain for the operator and eliminating the need for extra UV- or IR-blocking filters. A neutral color-balancing filter, typically NCB11, is often placed in the light path to adjust the color temperature of the incandescent light nearer to that of daylight, while LEDs are often pre-tuned to this color balance as a manufacturing specification without the need for an additional filter.
Other filters may be used to increase the visual contrast in cytogenetics specimens. For instance, contrast in G-banded chromosomes can be improved using a simple green glass filter that absorbs light of all colors except green. Depending on the correction level of the microscope optics, performance may be improved with monochromatic green light. In this case, a more efficient green interference filter is better at producing monochromatic green light for the best imaging conditions. Interference filters reject unwanted wavelengths by reflecting and causing destructive interference. A green interference filter (often labeled GIF) can be differentiated visually from a green glass filter by its unique reflective property, which often produces an orange or yellow tint when viewed at an angle. Interference filters, including those used for fluorescence, have very thin-layered coatings on their surfaces, and great care should be taken when cleaning them.
Field Diaphragm, Condenser, and Aperture Diaphragm
The field diaphragm, typically located after the light source and its associated filters, is an adjustable iris-type diaphragm that defines the total area of illumination. It should be opened just past the field of view, whether to the eye or camera sensor, to fully illuminate the specimen while reducing stray light. Proper Köhler illumination will focus the image of the field diaphragm in the specimen plane.
The microscope condenser gathers and focuses light from the source and passes it through the specimen, providing full and even illumination. The condenser assembly contains an adjustable diaphragm in its front focal plane known as the aperture diaphragm and may also house various light conditioners used in advanced contrasting techniques.
The maximum angle of incidence for light rays in the cone of light that the condenser can deliver is determined by the numerical aperture (NA) of the condenser. This optical property of lenses ultimately determines the resolving power of the optical system, which is the limit of its ability to separate fine details. While the objective lens may be the most prominent component that affects magnification and resolution, the effective NA (and thus resolving power) of the objective collecting transmitted light cannot exceed the NA of the condenser that delivers that light. For optimal resolution, the NA of the condenser should closely match or exceed the NA of objective lens.
The simplest common condenser is known as an Abbe condenser named for its inventor, Ernst Abbe. While Abbe condensers are available with a variety of NAs, they do not have significant correction for optical aberrations. The Abbe condenser can be used for basic inspection of routine brightfield samples but may not be suitable for critical or high-detail investigations, such as in cytogenetics.
Aplanatic condensers are corrected for spherical aberration, which is an optical imperfection characteristic of lenses with curved surfaces in which light rays passing near the lens periphery focus to a different point than rays traveling through the center of the lens, leading to a reduction in sharpness. Correction for this aberration may be accomplished for individual wavelengths of light. The performance of aplanatic condensers is best using green light, and this is assumed in the optical design since aplanatic condensers are not corrected for chromatic aberrations.
Chromatic aberration in microscopy generally refers to axial chromatic aberration, in which light of differing wavelengths does not focus to the same point. Achromatic condensers are corrected for axial chromatic aberrations to bring blue and red light to the same focus as green light, but they are not corrected for spherical aberration. Aplanatic-achromatic condensers are corrected for both spherical and chromatic aberrations.
The effective NA of the condenser can be adjusted by opening or closing the aperture diaphragm, thus increasing or reducing the angle of light entering the specimen and objective. Since higher NA relates to higher resolving power, it may seem counterintuitive to purposely reduce the NA of the condenser by restricting the diaphragm. However, as the aperture diaphragm is closed down and the NA is reduced, visual contrast and depth of field are both increased even as ultimate resolving power is decreased. Visual contrast lost by opening the aperture can often be restored by processing digital images. A clear understanding of this interplay between contrast and resolution helps the user navigate challenging samples and extract the maximum amount of information.
Objective Lenses
The objective lens is the major determinant of magnification and resolution and is perhaps the most significant single component of the optical train with regard to observational capability. Most manufacturers offer a wide selection of objectives with various magnifications and NAs and with varying optical design considerations for aberration correction and application. Objectives typically have markings on the barrel, indicating magnification, NA, aberration correction, working distance, immersion medium, and coverslip correction.
Resolution vs. Magnification
The two main functions of objectives are to resolve detail and magnify the image. It is a common misconception that magnification determines resolution when, in fact, the resolving power of a lens is a function of numerical aperture. The ability to resolve detail is directly tied to the numerical aperture of the objective such that higher NAs translate to higher resolving power, while magnification makes those resolved details easier to observe. Magnification increases size but not resolution.
As waves of light encounter very small objects, particularly of a size approaching the wavelength of the light, they are bent around the object. This property of light is described as diffraction. It can also refer to the way light waves spread as they pass through small apertures. As light is passed through a small object of interest on a microscope, such as a chromosome, the light that holds the information about that object may propagate at very steep angles relative to the optical axis. NA describes an objective’s ability to collect this highly diffracted light. The more highly diffracted the light that is collected, the better that object can be resolved by the lens. Since NA describes light-collecting ability, it also indicates relative brightness of the image produced, which is of major importance in light-challenged applications such as fluorescence.
The method of observation (visual vs. imaging system) should be considered when selecting magnification. For visual inspection, magnification selection is dependent on whether relevant resolved details can be observed by the human eye. For digital imaging, the required level of magnification needed to accurately record resolved details depends on the physical pixel size of the detector. In general, higher NAs call for smaller pixels, which are usually a consequence of a greater number of pixels on the detector, but this need is mitigated as magnification increases. The Nyquist sampling theorem states that to accurately record and reconstruct a continuous analog signal using discrete units (pixels in this case), the sampling rate, or frequency of the digital data point in time or space, must be at least two times the smallest observable signal.
A common rule of thumb for digital cameras is that effective pixel size in the specimen plane should be 2.5 times the maximum resolution of the objective. For example, to determine the resolution of an objective with a 1.3 NA, assuming long green light of 589 nm, the Rayleigh criterion for resolution (0.61λ/NA) gives a result of ∼276 nm for resolvable detail. Magnifying this result by 100× results in a resolved size at the detector of 27.6 mm. In order to sample 2.5 times per 27.6 mm, the physical pixel size of the detector must be smaller than 27.6/2.5 or ∼11 mm. However, if the magnification of the objective were only 40×, the resolved size at the detector is 276 nm × 40 or 11.04 mm, which would require a pixel size smaller than 11.04/2.5 or ∼4.4 mm.
Objective Types
Another major consideration in objective selection is the level of correction for optical aberrations. Common types of objectives include achromat, plan, fluorite, and apochromat. While the specific details of correction naming conventions are not completely standard across all manufacturers in the industry, these serve as a good general description of the type of optical corrections engineered in the lens.
Plan lenses are corrected for flatness of field so that the periphery of the field of view lies in the same focal plane as the center. Non-plan lenses may exhibit field curvature in which the edges of the image are out of focus, while the center is in focus. Flatness of field is very important for digital imaging, though in practice, even non-plan lenses may be flat in the field of view of the camera sensor, which is often smaller than the full visual field of view. The plan designation is often combined with other corrections, such as plan achromat, plan fluorite, or plan apochromat.
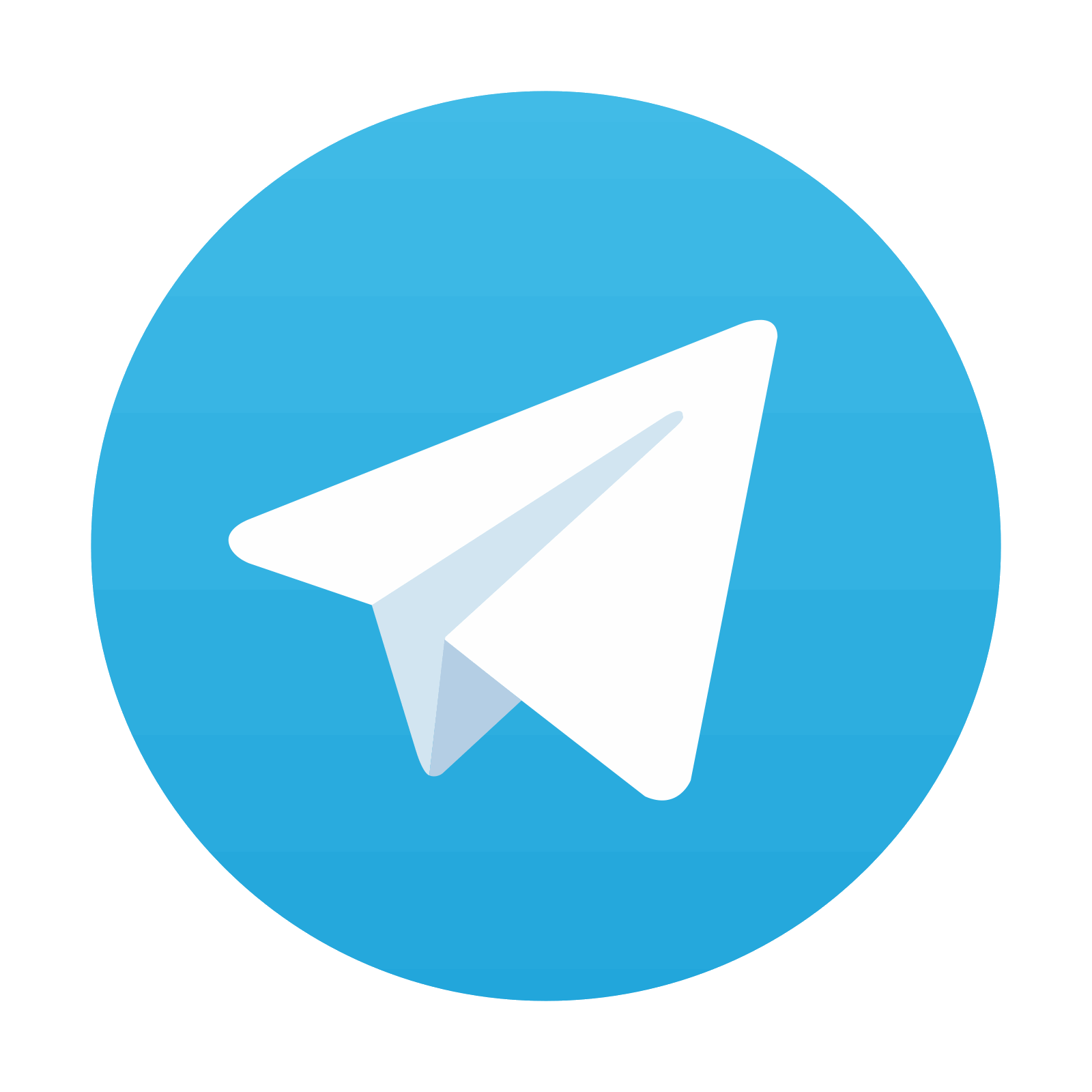
Stay updated, free articles. Join our Telegram channel
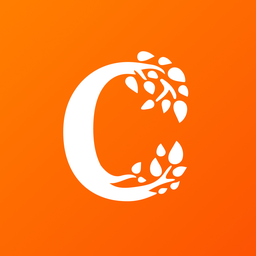
Full access? Get Clinical Tree
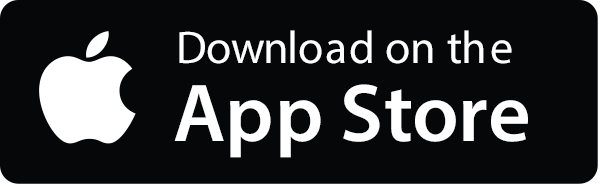
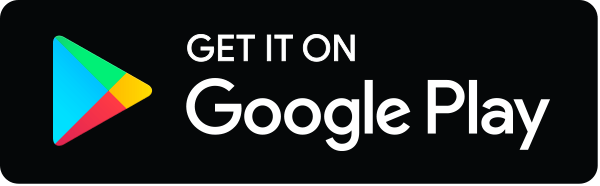