Fig. 9.1
Chromosome rearrangements can be produced by nonallelic homologous recombination between shared sequences or repeats of identical (direct repeats) or opposite (inverted repeats) orientation. Recombination between direct, nonallelic repeats on homologous chromosomes (a) or sister chromatids (b) can produce complementary duplications and deletions. Recombination between direct repeats located at different sites within a single chromatid can produce both deletions and acentric ring chromosomes (c). If instead recombination occurs between inverted repeats within a single chromatid, a chromosome inversion is produced (d). Translocations and other more complex rearrangements can occur secondary to recombination events between shared sequences that are located on different chromosomes (e). Shared sequences or repeats are designated by arrows and lower case letters represent unique sequences
The size of the inversions, duplications, and deletions produced by the recombination events described earlier is dependent upon the length and proximity of the LCRs mediating the rearrangement. In general, the larger the rearranged region, the larger the LCR that mediates the recombination event. Single gene rearrangements occur when the recombining homologous sequences flank or are within a single gene. These rearrangements are submicroscopic, require molecular techniques for their identification, and typically result in Mendelian genetic disorders such as Hunter syndrome, hemophilia A, familial juvenile nephronophthisis, and others [6, 9]. In contrast to single gene rearrangements, recombination events that utilize nonallelic homologous sequences that are separated by large regions of the genome (typically 1.5–5 Mb) or are located on different chromosomes altogether can produce cytogenetically visible rearrangements involving multiple genes. Included within this group are many of the recognized microdeletion and microduplication syndromes, as well as several recurring rearrangements such as the (4;8) translocation with breakpoints at 4p16 and 8p23 and the inverted duplicated chromosomes derived from chromosomes 15 and 22 (see respective sections later).
While LCRs appear to serve as the recombination substrates for many chromosomal rearrangements, high-copy-number repeats such as Alu or satellite DNA sequences also play a role. At least 32 cases of single gene disorders and 16 cases of cancer have been attributed to intrachromosomal Alu-mediated recombination events [10]. While much less common, interchromosomal Alu-Alu recombination events also appear to occur. This is evidenced by a report of an XX male who carried an XY translocation mediated by Alu repeats [11]. Additionally, interchromosomal nonallelic recombination events mediated by high-copy satellite DNA sequences and/or other adjacent repetitive sequences located within the short arms of the acrocentric chromosomes are hypothesized to be responsible for the formation of at least some of the Robertsonian translocations (see section “Robertsonian Translocations”, later). Recombination events mediated by satellite DNA sequences are also thought to be responsible for the recurring inversions involving the heterochromatic region within the proximal long arm of chromosome 9 [12].
That DNA architecture may create “hot spots” for chromosome rearrangements has been supported by studies addressing the recurring (11;22) translocation (see section “Reciprocal Autosomal Translocations”, later). The breakpoints involved in this translocation are not associated with regions of homology but rather with unstable AT-rich palindromic sequences (DNA sequences that contain two inverted regions complementary to each other) susceptible to double-stranded breaks that are repaired by a process referred to as nonhomologous end jointing (NHEJ). These palindromic sequences are predicted to form imperfect hairpin- or cruciform-shaped secondary structures susceptible to nucleases that produce double-stranded breaks, which are then ligated to form the resulting translocation. Short, typically 2–5 base pair regions of homology at, or in close proximity to, the double-stranded breaks are often, but not always, used to promote ligation.
Despite the fact that the breakpoints of a number of translocations have now been examined, palindromic sequences have rarely been identified. Among the few that have been identified, with one exception, the same AT-rich palindromic sequence within the proximal short arm of chromosome 22 employed in the recurring (11;22) translocation has been utilized [13]. Two reported cases involve independent (17;22) translocations mediated by a palindrome within intron 31 of the neurofibromatosis I (NFI) gene, at least five cases involve a recurring (8;22) translocation with nearly identical breakpoints within an AT-rich palindrome at 8q24.13, while the others involved palindromic sequences at 1p21.1 and 4q35.1 [13–18]. Most rearrangements formed secondary to NHEJ therefore do not appear to utilize palindromic sequences. Instead, they tend to occur within other areas of the genome that are predicted to form cleavage-sensitive chromatin structures vulnerable to the formation of double-stranded breaks such as topoisomerase II cleavage sites, DNase I-sensitive sites, scaffold attachment regions, or expanded trinucleotide repeat regions [1, 19]. Additionally, although the most thoroughly studied example of a rearrangement formed secondary to NHEJ is the recurring (11;22) translocation described earlier, most rearrangements that utilize this mechanism are nonrecurring. Examples of nonrecurring rearrangements suspected of forming primarily by NHEJ include the 1p36 and 11p11.2 deletions associated with 1p36 deletion and Potocki-Shaffer syndrome, respectively, the 10q24 duplications associated with split hand-split foot malformation [20], and others [7].
Recently, a third mechanism, fork stalling and template switching (FoSTeS), has been proposed to explain the formation of multiple complex nonrecurrent structural chromosome rearrangements that do not appear to have formed secondary to either NAHR or NHEJ [21, 22]. The complex rearrangements associated with FoSTeS contain duplications and/or deletions that are interspersed with nonduplicated or nontriplicated segments that are believed to form secondary to a promiscuous lagging replication strand that may not only have moved discontinuously within its own replication fork but may also have invaded other replication forks. This abnormal replication process is thought to be initiated by fork stalling secondary to particular DNA structures and/or protein-DNA complexes. Once stalled, the lagging DNA strand disengages from its original template and, using short regions of homology, reinitiates replication elsewhere within the same chromosome, the homologous chromosome, or a nonhomologous chromosome in close proximity. Whether the same or a different chromosome has been invaded, the location of strand invasion is upstream or downstream relative to the original replication fork, and whether the leading or lagging strand of the new replication fork has been invaded will dictate whether an interstitial or interchromosomal rearrangement has formed and whether genetic material has been duplicated in a direct or inverted orientation or has been completely deleted. The number of serial replication fork disengagements and invasions that occur prior to reestablishing normal replication on the original strand will dictate the complexity of the resulting rearrangement. Many of the PLP1 gene duplications associated with Pelizaeus-Merzbacher disease, as well as the nonrecurrent 17p11.2 duplications associated with Potocki-Lupski, are believed to occur secondary to FoSTes [23].
In contrast to the maternal bias noted for numerical chromosome abnormalities, approximately 75% of structural chromosome rearrangements appear to be paternally derived [24, 25]. Exactly why the male bias for de novo structural rearrangements exists is currently unknown. It has been suggested, however, that the lifelong mitotic proliferation of spermatogonial cells, compared to the finite number of mitotic divisions responsible for oögonial cell production in the female embryo, may promote the accumulation of mutations. Additionally, studies on mouse and drosophila suggest that male gametogenesis may be more sensitive to mutagens than oögenesis [26]. It is interesting to note, however, that although structural rearrangements as a group are more commonly paternal in origin, there are some exceptions to this rule. For example, approximately 90% of de novo nonhomologous Robertsonian translocations and 80% of terminal chromosome 1 short arm deletions are maternal in origin [27, 28]. Several supernumerary isochromosomes and inverted duplicated chromosomes also appear to form primarily during maternal gametogenesis [29–32]. No parental bias has been noted for several other types of rearrangements including the interstitial microdeletions associated with DiGeorge and Williams syndrome [33, 34]. Although the differences noted in male versus female gametogenesis are thought to affect our respective predispositions to producing specific types of de novo rearrangements, other factors, such as the effect of imprinting on fetal survival, have also been proposed to play a role (see Chap. 20).
In theory, chromosome breakage, rearrangement, and reunion can occur during meiosis or mitosis. Meiotic errors, since they occur prior to conception, would be expected to be present in every cell of the resulting pregnancy. Postconception mitotic errors, in contrast, would be predicted to produce a mosaic pregnancy containing both normal and abnormal cells. Interestingly, with the exception of mitotically unstable chromosomes such as rings or dicentrics, structural chromosome rearrangements are rarely seen in mosaic form. While this observation suggests that many structural rearrangements may be formed during meiosis, ascertainment bias likely plays a role as well. Since mosaic individuals typically have milder phenotypes than comparable nonmosaics, they are less likely to be ascertained and karyotyped. This would be especially true of individuals carrying mosaic balanced rearrangements. Additionally, mosaicism is difficult to detect, particularly when it is limited to a specific tissue or group of tissues, is present at a low level, and/or involves a subtle structural change.
Differentiating Between Balanced and Unbalanced Structural Rearrangements
Structural rearrangements are often divided into two general categories, balanced and unbalanced. Balanced rearrangements contain no net loss or gain of genetic information and the individuals who carry them are generally phenotypically normal. In contrast, additional and/or missing genetic material is present in individuals who carry unbalanced rearrangements. Just as modifications in the amount of the various ingredients added to any recipe cause changes in the final product, deviation from the normal disomic genetic complement results in a clinically affected individual.
While it is easy to define balanced and unbalanced rearrangements, distinguishing between a truly balanced and an unbalanced rearrangement using traditional cytogenetic techniques is often impossible. The maximum level of resolution obtained using standard microscopy of G-banded prometaphase chromosomes is reported to be 3–5 megabases or 3–5 × 106 base pairs. This number will vary, however, depending on the quality of the chromosome preparations and the skill of the cytogeneticist examining the karyogram(s). The ability to resolve or identify a rearrangement will also be influenced by the degree to which the banding pattern, overall size, and centromere location of an involved chromosome is altered. Obviously, the more apparent the change, the more likely it is to be detected. A number of molecular cytogenetic techniques such as fluorescence in situ hybridization (FISH), 24-color karyotyping, and chromosome microarray analysis are currently being used to detect submicroscopic or otherwise cryptic rearrangements that cannot be detected using traditional cytogenetics (see Chaps. 17 and 18). Recent studies suggest that chromosome imbalances can be found in an additional 10–14% of patients using chromosome microarray analysis [35–37]. Additionally, chromosome microarray analysis has demonstrated that as many as 40% of phenotypically abnormal individuals with apparently balanced simple chromosome rearrangements detected by traditional karyotyping actually contain submicroscopic gains and/or losses at or near one or more rearrangement breakpoints, while others have been found to have cryptic imbalances unrelated to their identified balanced rearrangements [38–41].
Associated Risks
Once a structural chromosome rearrangement is detected, regardless of whether it is balanced or unbalanced, the subsequent steps to take depend on the type of specimen that was analyzed. For prenatal samples or those obtained from a child, parental karyotypes or molecular cytogenetic studies should be obtained whenever possible to assess whether the rearrangement has been inherited or represents a de novo mutation. If neither parent is found to be a carrier of the rearrangement, the most likely scenario is that it represents a de novo abnormality rather than an inherited one. Non-paternity should be considered. Since the possibility of gonadal mosaicism can never be excluded, this family would be given a very low risk of having another child with the same structural abnormality. Prenatal testing would also be offered for all future pregnancies.
In contrast to the very low recurrence risk quoted to a couple with a child or pregnancy carrying a de novo rearrangement, the risk of chromosomally abnormal conceptions for an adult who carries a balanced structural rearrangement is much higher. In fact, for some familial rearrangements, the risk can approach 50%, and for very rare carriers of a homologous Robertsonian translocation, the risk for an abnormal conception is 100%. It is therefore imperative that these families are identified so that they can be given accurate genetic counseling regarding their reproductive risks and options. In situations where a familial rearrangement is identified, it must be remembered that it is not just the immediate family but distant relatives as well who may be at risk for having children with unbalanced karyotypes and associated mental and/or physical abnormalities. By systematically karyotyping the appropriate individuals in each generation, all those with elevated reproductive risks can be identified and appropriately counseled regarding their risks and options. Although there has been some debate regarding the appropriateness of karyotyping the phenotypically normal minors of balanced carriers, 50% of whom would be expected to be balanced carriers themselves, there is a consensus that these children should be referred for appropriate genetic counseling when they reach reproductive age.
The situation becomes a bit more complex when chromosome analysis of a bone marrow or tumor specimen results in an apparently balanced rearrangement, not associated with any particular neoplasm, in all cells examined. In these cases, it is imperative to ascertain whether such a rearrangement represents a patient-specific acquired change (which can then be monitored during treatment, remission, relapse, or any change in disease aggression) or a constitutional abnormality present from birth.
The reasons for this are twofold. First of all, from the point of view of the physician treating the patient, the presence of any acquired cytogenetic change is significant (see Chaps. 15 and 16). Equally as important in the long term, however, is establishing whether the chromosome rearrangement is familial and therefore has potential reproductive consequences for the extended family. While the potential familial reproductive issues are understandably not the primary concern of oncology patients nor their physicians, given the critical nature of their disease and the fact that cancer patients are often well beyond childbearing age themselves, this is an issue that should not be overlooked. Genetic counseling is covered in detail in Chap. 21.
De Novo Rearrangements
Every chromosome rearrangement was at one time a new or de novo rearrangement that carried the risks associated with an undefined entity. Children who carry unbalanced rearrangements, regardless of whether they represent new mutations or an unbalanced form of a familial rearrangement, almost inevitably demonstrate an abnormal phenotype. An imbalance is an imbalance regardless of how it arose.
In contrast, accurate predictions regarding the phenotype of a child or fetus that carries an apparently balanced de novo chromosome rearrangement are more difficult to make. In this situation, it is not known what has occurred at the molecular level within the rearrangement, and there are no family members with the rearrangement from whom inferences can be made. The risk for an abnormal phenotype is therefore always higher for an individual with an apparently balanced de novo rearrangement than for an individual who has inherited a similar rearrangement from a normal parent. Obviously, these individuals also carry a significantly higher risk for phenotypic abnormalities than their chromosomally normal counterparts. Several population studies have shown, for example, that the incidence of de novo apparently balanced rearrangements among the mentally retarded is approximately seven times that reported in newborns [42]. Apparently balanced de novo rearrangements detected at amniocentesis have also been associated with a risk for congenital abnormalities that is two- to threefold that observed within the general population [1].
A number of different mechanisms are thought to be responsible for the abnormal phenotypes observed in children with apparently balanced de novo rearrangements. One possibility is that the translocation is not truly balanced. As discussed earlier, structural rearrangements that appear balanced at the microscopic level may actually contain large duplications and/or deletions at the molecular level. Another possibility is that the rearrangement is “balanced,” but a break has occurred within a critical gene or its surrounding regulatory sequences such that the gene product or its expression is altered. This scenario has been demonstrated in several patients with Duchenne muscular dystrophy, for example [43]. A position effect, in which the expression of a specific gene or group of genes is altered when the chromosome segment containing them is moved to a different location, could also result in an abnormal phenotype. Such an effect has been demonstrated in several X;autosome translocation chromosomes in which inactivation seems to spread from the inactive X chromosome into neighboring autosomal segments. This phenomenon has been documented in drosophila and plants as well. Finally, the possibility that an individual’s abnormal phenotype may be completely unrelated to his or her rearrangement must always be examined. Other nonchromosomal genetic disorders, prenatal exposures, birth trauma, non-paternity, etc., must all be considered.
Familial Rearrangements
Balanced structural rearrangements may pass through multiple generations of a family without detection. When these families are ascertained, it is usually due to the presence of infertility, multiple spontaneous pregnancy losses, and/or clinically abnormal family members (Fig. 9.2). Meiotic events that result in cytogenetically unbalanced conceptions can explain the presence of all three occurrences within these families.


Fig. 9.2
A pedigree of a family in which a balanced Robertsonian (13;14) translocation is segregating. Multiple spontaneous abortions (see individuals II-2, III-2, and III-4), abnormal children (III-5), and infertility are frequently observed in families segregating a balanced rearrangement
During normal meiosis, homologous chromosomes pair utilizing a mechanism of formation thought to depend, at least in part, upon interactions between their shared sequences. Under normal circumstances, all 23 pairs of homologous chromosomes align themselves to form 23 paired linear structures or bivalents that later separate and migrate to independent daughter cells (see Chap. 2). In cells carrying structurally rearranged chromosomes, pairing cannot occur in a simple linear fashion. Instead, complex pairing configurations are formed in an attempt to maximize pairing between homologous regions that now differ with regard to their chromosomal location and/or orientation (see the later sections “Deletions,” “Duplications,” “Inversions,” “Reciprocal Autosomal Translocations,” and “Insertions”). Chromosome malsegregation and/or particular recombination events within these complex configurations can then lead to unbalanced conceptions, many of which never implant or are spontaneously lost during gestation.
Cytogeneticists are frequently asked to make predictions regarding a balanced carrier’s risk of producing an abnormal liveborn child. While this is a legitimate question, it is in practice very difficult to answer accurately. One source of difficulty is the fact that, with very few exceptions, each family’s rearrangement is unique. Therefore, unless a family is large and accurate information regarding the reproductive history and phenotype of each family member is available, typically no empiric data are available from which to obtain risk values. A second source of difficulty one encounters in assessing the reproductive risks associated with a particular balanced rearrangement is the breadth and complexity of the variables involved.
One important factor that is considered when assessing the reproductive risks of a carrier parent is the extent of imbalance demonstrated by the potential segregants. In general, the smaller the imbalance, the less severe the phenotype, and the more likely the survival. An additional rule of thumb is that the presence of excess genetic material is less deleterious than the absence of genetic material. Another variable to be considered is the quality of the genetic information involved. Some chromosomes, such as 16 and 19, are infrequently involved in unbalanced structural rearrangements. Presumably, this occurs because of the importance of maintaining a critical dosage for a gene or group of genes on these chromosomes. Conversely, imbalances involving other chromosomes such as 13, 18, 21, X, and Y appear to be more easily tolerated. In fact, a complete trisomy involving any of these chromosomes is survivable.
Each family’s reproductive history can also provide important clues regarding the most likely outcome for an unbalanced pregnancy. As might be expected, those families or individuals who have had a liveborn child or children with congenital abnormalities, especially when an unbalanced form of the familial rearrangement has been documented, are at highest risk for having unbalanced offspring. In families or individuals in whom multiple spontaneous abortions and/or infertility are noted, the risk for liveborn unbalanced offspring would be expected to be lower. In these families, it is assumed that the unbalanced conceptions are being lost very early as unrecognized pregnancies (infertility) or later during gestation. Interestingly, the sex of the carrier parent, in some cases, also influences the risk of having unbalanced offspring. In situations where a sex bias does exist, the female carrier invariably possesses the higher risk. Why male carriers appear to produce fewer unbalanced offspring than their female counterparts is not known. Perhaps fewer unbalanced segregants form during spermatogenesis relative to oögenesis, and/or the selective pressure against unbalanced gametes is greater in the male, and/or imprinting effects may cause the unbalanced embryos of male carriers to be less viable than those of their female counterparts. Male infertility may also play role [26, 44] (see Chap. 11).
On rare occasions, an abnormal phenotype is observed in an apparently balanced carrier of a familial rearrangement. While some of these cases may simply represent coincidental events, other possible explanations exist as well. Very rarely, abnormal offspring resulting from uniparental disomy, or the inheritance of both homologous chromosomes from a single parent, has been documented in the offspring of balanced translocation carriers [45] (see Chap. 20). Incomplete transmission of a partially cryptic rearrangement has also been observed in the abnormal offspring of a phenotypically normal carrier parent. Wagstaff and Herman, for example, describe a family in which an apparently balanced (3;9) translocation was thought to be segregating [46]. After the birth of two phenotypically abnormal offspring with apparently balanced karyotypes, molecular analysis demonstrated that the father’s apparently balanced (3;9) translocation was actually a more complex rearrangement involving a cryptic insertion of chromosome 9 material into chromosome 8. Abnormal segregation of this complex rearrangement led to a cryptic deletion of chromosome 9 material in one sibling and a duplication of the same material in the other.
Phenotypic discrepancies between child and parent may also be explained by the presence of a recessive allele that is inherited from a chromosomally normal parent. While the parent is phenotypically normal due to the presence of a complementary normal allele on the homologous chromosome, the abnormal allele can be expressed in the offspring, who has no normal allele. The affected child inherits two mutant alleles; one mutant allele is inherited secondary to the balanced chromosome rearrangement, while the other is inherited from the cytogenetically normal parent (Fig. 9.3). In a slight variation of this theme, the second inherited hit or mutation in the affected child is nonallelic but presumably functions within a biological pathway identical to or related to the first hit. The idea is that each parent carries a single mutation that is benign or causes only mild clinical manifestations of disease. When both mutations are inherited together, however, the involved pathway(s) is sufficiently altered to cause disease. Alternatively, one predisposing mutation could be inherited, while the other occurs de novo.


Fig. 9.3
In the example here, the mother (top left) carries a recessive point mutation (asterisk) within a gene (black box) located on one chromosome homolog (hatched). The father (top right) carries a mutation in the same gene secondary to interruption via a translocation event. Because the second homolog in each parent contains a normal allele, both parents are phenotypically normal. This is also true for their first child (bottom left) who inherited the balanced translocation from her father and the normal hatched chromosome from her mother. Although their second child (bottom right) is also a balanced translocation carrier, she has inherited two mutated copies of the gene and therefore manifests the recessive disease. The allele she inherited from her mother contains a point mutation, while the comparable paternally inherited allele has been interrupted secondary to a translocation
The two-hit scenario described earlier has also recently been invoked to provide one possible explanation for the variable expressivity and decreased penetrance observed in association with many microdeletions and microduplications. This is especially true of some of the smaller copy number changes that are currently being identified by chromosome microarray analysis [47, 48].
Deletions
Autosomal deletions that can be detected by traditional, “high-resolution,” or molecular cytogenetic methods produce monosomies that are generally associated with significant pathology. Some exceptions, however, do exist. Loss of the short arm material from acrocentric chromosomes during the formation of Robertsonian translocations, for example, has no impact on phenotype. Similarly, the striking size variation of heterochromatic regions in normal individuals suggests that loss of some, if not all, of this material is insignificant. There have even been reports of “benign” deletions in regions that are considered euchromatic. Gardner and Sutherland catalog deletions of this type in bands 2p11.2-p12 and 2q13-q14.1; 3p25.3-pter; 5p14; 8p23.1-pter and 8q24.13-q24.22; 9p21.2-p22.1; 11p12; 13q21; 16q21 and 16q13-q22; and 18p11.2-pter [49]. Close examination of these regions reveals that many are relatively gene poor and therefore less likely to contain a dosage-sensitive gene. For example, the 11 Mb of genomic material within the 5p14 band and the 7 Mb of genomic material within the 11p12 band contain only eight and nine genes, respectively.
Among deletions of pathological significance, classic cytogenetic deletions that can be detected by routine methodology tend to be larger and associated with major malformations. Generally, large deletions have a more significant impact on phenotype and survival than smaller ones. The nature of the deleted material, however, also plays an important role in determining whether a specific deletion is viable. Thus, deletions of large segments of the short arms of chromosomes 4 and 5, and of the entire short arm of chromosome 18, are recurrent abnormalities among infants with major malformations, while deletions of similar size involving the short arms of chromosomes 17 and 19 are rarely, if ever, seen in liveborns [50].
Classic deletions have traditionally been described as either terminal (Fig. 9.4) or interstitial (Fig. 9.5) based on chromosome banding patterns. A deletion is considered “terminal” if there is no discernable material beyond the site of initial breakage. Conversely, interstitial deletions have a proximal breakpoint, missing material, and a more distal breakpoint beyond which the chromosome continues with a normal banding pattern to its terminus. All stable chromosomes have telomeres comprised of the human consensus telomere sequence (TTAGGG)n. Chromosomes with apparent terminal deletions are no exception and are assumed to have acquired “new” telomeres following the deletion event.



Fig. 9.4
A terminal deletion involving the distal short arm of chromosome 5 [del(5)(p15.3)]. Patients with similar deletions are said to have cri du chat or cat cry syndrome because of the characteristic catlike cry present in many during infancy

Fig. 9.5
An interstitial deletion involving the long arm of chromosome 13 [del(13)(q21.3q33)]
Several mechanisms for acquiring or retaining a telomere have now been documented among chromosome deletions. One mechanism referred to as telomere healing involves the addition of a new (TTAGGG)n sequence at or near the deletion breakpoint [51–53]. In these cases, a telomerase recognition site in the vicinity of the deletion breakpoint is bound by the enzyme telomerase, which synthesizes a completely new telomere. These therefore represent true terminal deletions. Other chromosomes with apparent terminal deletions have been shown to actually represent derivative chromosomes that have acquired their subtelomeric and telomeric regions from another chromosome secondary to a translocation event. These translocation or “telomere capture” events are hypothesized to occur secondary to homologous recombination mediated by regions of shared homology that exist within the deleted chromosome and the subtelomeric region of a separate chromosome [54, 55]. Still other deletions appear to be terminal by traditional cytogenetic analysis but have been shown by molecular analysic analysis to be interstitial. It is estimated that 7–25% of apparent terminal deletions fall into this category [56–58]. Because a chromosome with an interstitial deletion retains its original telomere, there is no reason to synthesize or acquire a new one.
The use of “high-resolution” banding and molecular cytogenetic techniques has led to the identification of another class of cytogenetic abnormality variously referred to as chromosomal microdeletions, contiguous gene syndromes, and segmental aneusomy syndromes (SAS). These abnormalities are mostly very small interstitial deletions, often at or below the resolution of microscopic analysis, that recur with appreciable frequency and are associated with distinct clinical phenotypes. The term, “microdeletion,” is descriptive but fails to include the minority category of “microduplications” (e.g., CMT1A; see also section “Duplications,” later) and the variable etiologies for some of the disorders. The term “contiguous gene syndromes” was introduced in 1986 to describe the involvement of multiple contiguous genes in the production of a clinical phenotype [59]. While this terminology remains appropriate for some of the disorders in this new category, others are actually single gene disorders, or the result of imprinting defects or uniparental disomy (see Chap. 20). In an effort to more accurately characterize the pathogenesis of these disorders, the term segmental aneusomy syndrome was proposed to imply that the phenotype is the result of “inappropriate dosage for a critical gene(s) within a genomic segment” [60].
Williams syndrome is one example of an SAS that results from a small deletion [61]. These patients typically carry a ∼1.5 Mb deletion within the proximal long arm of chromosome 7 that encompasses approximately 30 different known and predicted genes. At least some of these genes appear to be responsible for the cardiovascular abnormalities, growth and developmental delays, infantile hypercalcemia, and dysmorphic facial features that are associated with Williams syndrome. Deletion of the elastin gene (ELN), for example, has been implicated in the cardiovascular abnormalities. This gene is also presumed to play a causative role in some of the other features associated with this syndrome including renal artery stenosis, hypertension, hoarse voice, premature sagging of the skin, and perhaps some of the facial features. Similarly, loss of LIM-kinase 1 (LIMK1), a novel kinase expressed in the brain, is predicted to explain some of the cognitive abnormalities in these patients. Presumably, some, or all, of the remaining genes identified within the common Williams syndrome deletion also contribute to the physical features associated with this contiguous gene syndrome.
Molecular studies of the Williams syndrome deletions have revealed the presence of flanking low-copy repeat (LCR) sequences at the common breakpoint sites. These LCR sequences appear to provide recombination sites for unequal meiotic and mitotic exchange events that produce the recurring Williams syndrome deletions [62–64]. In some cases, these unequal exchange events seem to be promoted by the presence of heterozygosity for a submicroscopic paracentric inversion that spans the same low-copy repeat sequences that mediate the common 1.5 Mb Williams syndrome deletion [65–68]. It is estimated that the risk of having a child with a Williams syndrome deletion is approximately fivefold higher for an individual who is heterozygous for this inversion when compared to an individual who does not carry it. However, despite their elevated relative risk, it is important to note their absolute risk remains low at approximately 1 in 1,750. Several studies have now demonstrated that, at least in some cases, these inversions increase the probability of a rearrangement by producing better LCR substrates for recombination. In the case of the recurring 15q13.3, 16p12.1, and 17q21.31 microdeletions, for example, the associated inversion polymorphism increases the probability of an unequal exchange by changing the directional relationship between the mediating LCRs [48, 69, 70]. Without the inversion polymorphism, the LCRs are in an inverted orientation and unequal exchange is not promoted. With the inversion, however, the LCRs are placed in a direct orientation and as such are ideally suited for the NAHR events between homologous chromosomes and sister chromatids that are primarily responsible for these deletions (Fig. 9.1a, b). In other cases, the inversion may improve upon the involved LCR substrates by increasing their length. It is also possible that the inversion loop that forms to maximize homologous chromosome pairing in the heterozygous parent renders the paired chromosomes more susceptible to unequal crossing-over (see section “Inversions” and Fig. 9.12 later).
As noted for Williams syndrome, flanking LCR sequences have also been found at the deletion sites of several other SASs. Recombination events localized to these LCR sequences appear to account for the size consistency and the frequency of the deletions associated with these disorders as well. A partial listing of classic cytogenetic deletion or SASs can be found in Table 9.1. Given the recent widespread use of chromosome microarray technology in many research and clinical cytogenetics laboratories, the number of microdeletion and microduplication syndromes being identified and characterized is growing at a rapid pace. For an extensive list of chromosome abnormalities that includes some of the more recently indentified syndromes and their associated phenotypes, see the Websites for Wellcome Trust Sanger Institute “DECIPHER,” the European Cytogeneticists Association Register of Unbalanced Chromosome Aberrations (ECARUCA), and Unique – The Rare Chromosome Disorder Support Group [71–73].
Table 9.1
Some recurring deletion syndromes
Deletion syndrome | Deleted region | Key clinical features |
---|---|---|
Monosomy 1p36 | 1p36a | Mental retardation, growth delay, hypotonia, early puberty, deafness, eye problems, cardiomyopathy, seizures and/or abnormal EEGs, enlarged anterior fontanel, deep-set eyes, flat nasal bridge, orofacial clefting or palatal abnormalities, pointed chin, ear abnormalities |
Wolf-Hirschhorn | 4pa | Mental and growth retardation, microcephaly, hypertelorism, broad nasal bridge, downturned mouth, cleft lip and/or palate, micrognathia, cryptorchidism, hypospadias |
Cri du chat | 5pa | Mental and growth retardation, cat-like cry in infancy, microcephaly, round face, hypertelorism, down-slanting palpebral fissures |
Sotos | 5q35b | Cardinal features include intellectual disability, overgrowth, and characteristic long, thin facies with a broad forehead, sparse frontoparietal hair, and down-slanted palpebral fissures. Macrocephaly, advanced bone age, behavior problems, hypotonia, feeding problems, renal anomalies, scoliosis, and seizures are also seen |
Williams | 7q11.23b | Mental retardation, short stature, supravalvular aortic stenosis, hypercalcemia, friendly disposition, hoarse voice, periorbital fullness, stellate pattern in the iris, anteverted nares, long philtrum, full lips |
Potocki-Shaffer (DEFECT 11 syndrome for deletion, enlarged foramina, exostoses, cranial dysostosis, retardation) | 11p11.2a | Mental retardation, biparental foramina, brachycephaly, turricephaly, multiple exostoses, micropenis, and minor facial dysmorphism including a high forehead, small upturned nose with broad tip, downturned mouth |
Jacobsen | 11q24.1–11qtera | Mental and growth retardation, trigonocephaly, strabismus, cardiac defects, digit anomalies, thrombocytopenia |
Langer-Giedion (trichorhinophalangeal syndrome type II) | 8q24.11–8q24.13a | Mental and growth retardation, multiple exostoses, cone-shaped epiphyses, fine scalp hair, bulbous nose, prominent ears, simple but prominent philtrum, loose redundant skin in infancy |
Angelman | Maternal 15q11.2–15q13.1 deletion complementary to the 15q11.2–15q13.1 microduplication syndrome | Mental and growth retardation, frequent laughter, ataxia and jerky arm movements, seizures, maxillary hypoplasia, deep-set eyes, large mouth with protruding tongue, widely spaced teeth, prognathia |
Prader-Willi | Paternal 15q11.2–15q13.1 | Mental and growth retardation, hypotonia and feeding problems in infancy, later obesity associated with hyperphagia, narrow bifrontal diameter, almond-shaped eyes, small hands and feet, hypogonadism, skin picking |
15q13.3 Microdeletion | 15q13.3b | Developmental delay with mild to moderate learning disability, autism spectrum disorder, schizophrenia, epilepsy, seizures, digit anomalies, and facial features that include hypertelorism, short philtrum, and a thick, everted upper lip. Extensive phenotypic variability and incomplete penetrance have been reported |
Rubinstein-Taybi | 16p13.3b | Mental retardation, postnatal growth retardation, hypotonia, broad thumbs and toes, cryptorchidism, abnormal facies with downward-slanting palpebral fissures; heavy, highly arched eyebrows; long eyelashes; prominent and/or beaked nose; hypoplastic maxilla with narrow palate |
Miller-Dieker | 17p13.3a | Mental and growth retardation, lissencephaly, microcephaly, bitemporal depression, long philtrum, thin upper lip, mild micrognathia, ear dysplasia, anteverted nostrils |
Hereditary neuropathy with liability to pressure palsies (HNPP) | 17p11.2b | Asymmetric recurrent palsies precipitated by focal pressure beginning in the second or third decade of life and electrophysiologic findings of prolonged sensory motor nerve conduction |
Deletion complementary to the CMT1A syndrome duplication | ||
Smith-Magenis | 17p11.2a | Mental retardation, behavioral problems, hyperactivity, sleep disturbance, decreased pain sensitivity, short stature, brachycephaly, midface hypoplasia, prognathism, fingertip pads, hoarse voice |
17q21.3 Microdeletion | 17q21.3b Deletion complementary to the 17q21.2 microduplication syndrome | Mental retardation/developmental delay, delayed speech, friendly disposition, hypotonia, normal growth, epilepsy, heart anomalies, renal/urologic anomalies, abnormal hair color or texture, and typical facies with high broad forehead, ptosis, blepharophimosis, up-slanting palpebral fissures, epicanthal folds, a tubular- or pear-shaped nose, prominent ears |
Alagille | 20p12.2b | Cholestasis, peripheral pulmonic stenosis, vertebral arch defects, posterior embryotoxon, abnormal facies including deep-set eyes, broad forehead, long straight nose, prominent chin, small low-set or malformed ears |
DiGeorge/velo-cardio-facial (Shprintzen) | 22q11.2aDeletion complementary to proximal 22q11.2 microduplication syndrome | Learning disabilities, short stature, overt or submucous cleft palate, velopharyngeal incompetence, prominent nose with squared nasal root and narrow alar base, conotruncal cardiac defects, and psychiatric disorders in some |
Phelan-Mcdermid | 22q13.3a | Moderate to severe developmental delay, severe expressive speech delay, behavior disturbance, increased tolerance to pain, hypotonia, normal to accelerated growth, dysplastic toenails, large hands, and minor dysmorphic features including dolichocephaly, ptosis, abnormal ears, pointed chin |
Kallmannc | Xp22.3b | Hypogonadotropic hypogonadism, eunuchoid habitus, anosmia or hyposmia, bimanual synkinesia |
Ichthyosis (X-linked)c | Xp22.3b | Hypertrophic ichthyosis, corneal opacities without impairment of vision |
In contrast to the size consistency and recurrent use of specific LCR sequences documented among many of the interstitial SAS deletions, other deletions appear to have multiple independent breakpoints and vary considerably in size. This size variability has been noted in association with multiple deletions including those that involve the short arms of chromosomes 1, 4, and 5 [28, 74, 75]. Nonhomologous end joining (NHEJ) and fork stalling template switching (FoSTeS), rather than nonallelic homologous recombination (NAHR), are believed to be responsible for these nonrecurring rearrangements [7, 76, 77].
Duplications
The term “duplication” as applied to chromosome abnormalities implies the presence of an extra copy of a genomic segment resulting in a partial trisomy. A duplication can take many forms. It can be present in an individual as a “pure duplication,” uncomplicated by other imbalances (Fig. 9.6), or in combination with a deletion or some other rearrangement. Examples of some types of rearrangements that involve duplications include isochromosomes, dicentrics, derivatives, recombinants, rings, and markers. The origins and behavior of these abnormal chromosomes are discussed elsewhere in this chapter.


Fig. 9.6
A duplication involving the distal long arm of chromosome 15 [dup(15)(q24q26.3)]. This duplication was initially observed in the bone marrow of a patient with mental retardation and leukemia. By obtaining a peripheral blood karyotype, it was possible to demonstrate that the duplication was constitutional and apparently unrelated to the leukemia
Tandem duplications represent a contiguous doubling of a chromosomal segment. The extra material can be oriented in the same direction as the original (a direct duplication) or in opposition (an inverted duplication). Most cytogenetically detectable tandem duplications in humans appear to be direct [78].
Autosomal duplications produce partial trisomies and associated phenotypic abnormalities. As mentioned in the introduction, the phenotypes associated with duplications are typically less severe than those associated with comparable deletions. Relatively few duplications, however, have occurred with sufficient frequency or been associated with such a strikingly characteristic phenotype that they have been recognized as defined clinical syndromes (Table 9.2). A few cases of distal 3q duplication have been reported in patients with features similar to Cornelia de Lange syndrome. However, these patients also have additional abnormalities not usually associated with the syndrome [50]. Paternally derived duplications of distal 11p have also been associated, in some cases, with Beckwith-Wiedemann syndrome [79]. More intriguing, and perhaps more significant, is the emerging recognition of recurring duplications that involve the same genomic segments that are associated with some of the established microdeletion syndromes. These complementary microduplication/microdeletion syndromes are thought to represent the reciprocal products of recurring unequal exchange events that are mediated by flanking homologous low-copy repeat (LCR) sequences. The causative unequal exchange events can occur following misalignment of either sister chromatids or homologs as shown in Fig. 9.1.
Table 9.2
Some recurring duplication/triplication syndromes
Duplication/triplication syndrome | Duplicated/triplicated region | Key clinical features |
---|---|---|
Duplication 3q | ?3q26.3 | A Cornelia de Lange-like phenotype that includes mental retardation postnatal growth retardation, long philtrum, palate anomalies, anteverted nares, clinodactyly, talipes, renal and cardiac abnormalities |
7q11.23 Microduplication | 7q11.23b Duplication complementary to the 17q21.2 microdeletion syndrome | Cognitive abilities range from normal to moderate mental retardation, but all have speech delay. Other features include autism, hypotonia, heart defects, diaphragmatic hernia, cryptorchidism, and dysmorphic facial features including a short philtrum, thin lips, and straight eyebrows |
Beckwith-Wiedemann | 11p15.5b (Paternal) | Macrosomia, macroglossia, organomegaly, omphalocele, ear creases, hypoglycemia, tumor susceptibility. Beckwith-Wiedemann patients with cytogenetic duplications are more likely to have learning difficulties |
Pallister-Killian | Mosaic tetrasomy 12p usually secondary to an extra metacentric isochromosome | Mental retardation, streaks of hyper- and hypopigmentation, sparse anterior scalp hair, sparse eyebrows and eyelashes, prominent forehead, protruding lower lip, coarsening of face with age |
Proximal 15q11.2 microduplication | 15q11.215q13.1a Complementary to Prader-Willi/Angelman syndrome deletion region | Mild to severe intellectual impairment particularly with regard to language, autism spectrum disorders, decreased motor coordination, hypotonia, reduced deep tendon reflexes, joint laxity, mild or no dysmorphic features. Phenotype typically associated with maternal (but not paternal) duplication |
Pseudodicentric 15 (“inverted duplicated 15”) | Tetrasomy 15pter–15q13 due to the presence of an extra pseudodicentric chromosome | Mental and growth retardation, autism, behavioral disturbance, seizures, low posterior hairline, epicanthal folds, low-set ears, strabismus |
The smaller pseudodicentric 15 chromosomes may not cause phenotypic abnormalities | ||
17p13.3 Duplication | 17p13.3 Most are unique nonrecurrent duplications that overlap with but do not correspond to the recurring Miller-Dieker syndrome deletion region | Mental retardation and/or learning difficulties, speech difficulties, autism, hypotonia, subtle hand and foot malformations, lack of severe congenital anomalies, and normal to increased growth parameters. Facial features include prominent forehead and pointed chin |
Charcot-Marie-Tooth 1A (CMT1A) | 17p11.2b Duplication complementary to the HNPP syndrome deletion | Abnormal nerve conduction velocities, distal muscle weakness, muscle atrophy, and sensory loss. Symptoms begin between ages 5 and 25 and progress slowly |
Potocki-Lupski | 17p11.2a Duplication complementary to the Smith-Magenis syndrome deletion | Mild to borderline mental retardation, behavioral problems, hypotonia, failure to thrive, cardiac anomalies, and variable dysmorphic features that include triangular face, frontal bossing, microcephaly, hypertelorism, wide nasal bridge, epicanthal folds, and a flat philtrum |
17q21.3 Duplication | 17q21.3 b Duplication complementary to the 17q21.3 microdeletion syndrome | Intellectual disability, autism spectrum disorders, and variable dysmorphic features |
Proximal 22q11.2 microduplication | 22q11.2a Duplication complementary to the DiGeorge syndrome deletion | Currently no clearly established phenotype recognized. Some patients noted to have features that overlap with DiGeorge syndrome including mental retardation and developmental delay, abnormalities of the palate, conotruncal heart defects, absent thymus, and corresponding T-cell deficiency. Phenotype variable and ranges from mild to severe |
Cat eye | Tetrasomy 22q11.2 (occasionally trisomy) usually secondary to an extra pseudodicentric or ring chromosome | Usually mild mental retardation, coloboma of the iris, down-slanting palpebral fissures, preauricular tags and/or fistulas, anal atresia |
One of the first complementary microduplication syndromes identified involves the Prader-Willi and Angelman syndrome (PWS/AS) region within the proximal long arm of chromosome 15 (Fig. 9.7). These duplications are mediated by the same LCRs and encompass the same loci that are deleted in PWS/AS. The clinical significance of these particular duplications was initially difficult to assess because many affected patients had phenotypically normal relatives with the same apparent duplication. Molecular studies have now provided us with an explanation for the apparent absence of a genotype-phenotype correlation in these families. With few exceptions, the clinically affected individual(s) within these families demonstrate mental retardation, decreased motor coordination, autism spectrum disorder, and mild to no dysmorphic features and carry a maternally derived duplication. In contrast, the duplicated chromosome in the normal relatives of these patients is typically paternally inherited. These data suggest that imprinting within the PWS/AS region is responsible not only for the phenotypic differences we observe with maternal versus paternal deletions but also for the presence or absence of a clinical phenotype in patients with a duplication (see Chap. 20).


Fig. 9.7
A duplication involving the proximal long arm of chromosome 15 [dup(15)(q11.2q13.1)] that appears to be complementary to the common deletions that are observed in Prader-Willi syndrome and Angelman syndrome patients. Duplication of the Prader-Willi/Angelman syndrome region was confirmed using fluorescence in situ hybridization (not shown.)
In addition to the PWS/AS region, complementary microdeletion-microduplication syndromes have also been documented for the Williams, Smith-Magenis, 17q21.31, and DiGeorge syndrome critical regions, as well as for the hereditary neuropathy with liability to pressure palsies (HNLPP)/Charcot-Marie-Tooth type I region (Table 9.2). More recently, chromosome microarray analysis has resulted in the identification of additional previously unrecognized complementary recurring microdeletions and duplications involving 1q21.1, 3q29, 15q13.3, 16p11.2, 16p13.11, 17q12, distal 22q11.2, and multiple other regions [48]. For an extensive list of chromosome abnormalities that includes some of the more recently indentified microduplication and microdeletion syndromes and their associated phenotypes, see the Websites listed earlier in the section “Deletions” [71–73].
Inversions
Inversions are intrachromosomal rearrangements formed when a chromosome breaks in two places and the material between the two breakpoints reverses orientation. Inversions can be of two types: pericentric or paracentric. In pericentric inversions, the breakpoints lie on either side of the centromere and formation of the inversion often changes the chromosome arm ratio (centromere position) and alters the banding pattern of the chromosome (Figs. 9.8 and 9.9). Paracentric inversions, on the other hand, have both breakpoints on the same side of the centromere, or within a single chromosome arm (see Chap. 10, Fig. 10.6). In paracentric inversions, the centromere position does not change, and the only clue to their presence is an alteration in the chromosome banding pattern. Prior to the development of banding techniques, the existence of paracentric inversions was theorized but could not be proven.



Fig. 9.8
This benign inversion of chromosome 9 [inv(9)(p11q13)] represents a pericentric inversion with breakpoints in both chromosome arms. The material between the two breakpoints has been inverted, the block of heterochromatin that normally sits in the long arm has been shifted to the short arm, and the banding pattern has been subtly changed. Because the breakpoints have not occurred symmetrically with respect to the centromere, the short arm-to-long arm ratio of the inverted chromosome has been altered as well

Fig. 9.9
Although this recurring pericentric inversion [inv(2)(p11q13)] is considered to be benign, individuals who carry this inversion might have a slightly increased risk for miscarriages
In those studies in which parents of a proband with a unique inversion have been karyotyped, the inversion is found in a parent as often as 85–90% of the time [80–82]. Most inversions of both types therefore appear to be inherited. Additionally, among the handful of apparent recurring inversions studied thus far, most have not been formed from multiple independent events but instead have been inherited from a single common distant ancestor. Examples include inv(3)(p25q21), inv(5)(p13q13), inv(8)(p23q22), inv(10)(p11.2q21.2), and inv(11)(q21q23). The recurrent variant inv(2)(p12q13), however, appears to be one of the few exceptions (Fig. 9.9). This inversion has occurred in the human genome on multiple occasions, and its recurring formation appears to occur secondary to nonallelic homologous recombination (NAHR) [83, 84].
Pericentric Inversions
As discussed earlier, both recurring and unique pericentric inversions have been reported in man. Some recurring inversions are considered normal variants. In some of these variant inversions, a block of heterochromatin normally situated in the proximal long arm of the chromosome is inverted into the short arm of the chromosome. Such inversions are found in chromosomes 1, 9 (Fig. 9.8), and 16. A second group of apparently benign recurring inversions, which have breakpoints very near the centromere in both the long and short arms, are found in chromosomes 2, 3 and 10, and in the Y chromosome. These variant forms have been observed in a large number of families and appear to segregate without deleterious effect. One group of investigators, however, has reported an increased risk for miscarriage among carriers of a pericentric inversion of chromosome 2 [inv(2)(p11q13), Fig. 9.9] [85]. Other inversions have been observed in many families but are not without consequence. Of particular note is the inversion of chromosome 8 with breakpoints at p23 and q22, which has been seen in families of Mexican-American descent [86].
Unique inversions are those observed in a single individual or family. The clinical significance of these inversions must therefore be determined on a case-by-case basis; as described later, some inversions can impart substantial reproductive risk, depending on the chromosome segment involved.
Excluding the variant inversions discussed previously, the frequency of pericentric inversions in the human population has been estimated at 0.12–0.7% [49].
Meiotic Behavior and Risks for Carriers of Pericentric Inversions
In order to understand the reproductive risks of an inversion carrier (heterozygote), the meiotic behavior of inverted chromosomes must first be considered. In meiosis, homologous chromosomes pair in close association. During this pairing phase, genetic information is exchanged between homologs through a process known as crossing-over or recombination (see Chap. 2). Crossing-over appears to be a necessary step for orderly chromosome segregation and is the mechanism that ensures human genetic individuality. A chromosome pair that consists of one normal chromosome and one chromosome with an inversion cannot achieve the intimate pairing of homologous regions necessary for normal meiosis through simple linear alignment. The classic model for pairing in an inversion heterozygote is the inversion or reverse loop demonstrated in Fig. 9.10a. In this model, the inverted segment forms a loop that can then pair with homologous regions on the normal chromosome. The noninverted portions of the chromosome (the chromosome segments distal to the inversion breakpoints) pair linearly with homologous regions on the normal chromosome. An odd number of crossovers between the same two chromatids within the inversion loop will result in the production of recombinant chromosomes, while an even number of crossovers between the same two chromatids within the inversion loop should result in the production of normal or balanced chromosomes.


Fig. 9.10
Several models for meiotic pairing in a pericentric inversion heterozygote. (a) An inversion loop containing a single crossover and the resulting parental and recombinant chromosomes. Note that only the material that is distal to the inversion breakpoints has been duplicated/deleted in each recombinant chromosome. (b, c) Alternate models for pairing during which only partial pairing or synapsis occurs
Two types of recombinant chromosomes are formed when crossing-over occurs between the inversion breakpoints. One recombinant will contain a duplication of the material distal to the breakpoint on the short arm and a deletion of the material distal to the breakpoint in the long arm. The second recombinant is complementary to the first and contains a short arm deletion and a long arm duplication (Figs. 9.10 and 9.11). Both recombinants are known as duplication-deficiency chromosomes.


Fig. 9.11
Left: a normal chromosome 5 and a chromosome 5 with a large pericentric inversion, with breakpoints at p15.33 and q33.1, observed in a parent (* 5p terminal material, * 5q terminal material). Middle: a normal chromosome 5 and a recombinant chromosome 5 [rec(5)dup(5q)inv(5)(p15.33q33.1)] resulting from recombination within the inversion loop of the parental inversion carrier. This duplication-deficiency chromosome is missing the short arm material that lies distal to the short arm inversion breakpoint and has two copies of the long arm material positioned distal to the long arm inversion breakpoint. Right: a metaphase hybridized with subtelomere probes for the short arm (green) and long arm (red) of chromosome 5. Note that the recombinant or duplication-deficiency chromosome (arrowed) has two red long arm subtelomere probe signals and no green short arm subtelomere probe signal
Alternate models for pairing in an inversion heterozygote are seen in Fig. 9.10b, c. In inversions with very small inverted segments (breakpoints are close to the centromere and the distal segments are large), the noninverted segments of both chromosomes may pair in linear fashion, with asynapsis or failure to pair in the small inverted segment. In this model, crossing-over can only take place in the noninverted segments of the chromosomes, and thus abnormal recombinant chromosomes are not formed. In the opposite situation, where the inverted segment is very large relative to the size of the entire chromosome and the distal segments are small, pairing may occur only between the inversion breakpoints, and the distal material will remain unpaired. In this situation, a crossover between the inversion breakpoints would produce recombinant chromosomes in a manner similar to the reverse loop model discussed previously. Crossing-over could not take place in the segments distal to the inversion breakpoints since those regions do not pair.
Careful examination of the recombinant chromosomes produced when crossing-over takes place between the breakpoints in a pericentric inversion reveals that the genetic imbalance always involves the material distal to the inversion breakpoints. Thus, large inversions have small distal segments and produce recombinant chromosomes with small duplications and deficiencies, while small inversions have large distal segments and produce recombinant chromosomes with large duplications and deficiencies. In general, then, large inversions are associated with a greater risk of producing abnormal liveborn offspring since the recombinant chromosomes associated with them carry small duplications and deficiencies that have a greater probability of being compatible with survival. Furthermore, the larger the inversion, the greater the likelihood that a recombination event within the inversion loop will occur and form recombinant chromosomes. The opposite is true of small inversions with large distal segments, which are usually associated with a very low risk of liveborn abnormal offspring.
In addition to the size of the inverted segment, other factors must be considered when determining the reproductive risk associated with any given pericentric inversion. Since monosomies are generally more lethal than trisomies, an inversion that produces a recombinant with a very small monosomy may be associated with a relatively high risk of abnormal offspring.
The nature of the genetic material in the inverted chromosomes can also be important. For instance, both trisomy and partial monosomy of chromosomes 13, 18, and 21 are seen in liveborn infants with birth defects and mental retardation. Once the duplications and deficiencies associated with the recombinants from a particular inversion are identified, review of the medical literature for evidence that these duplications and/or deficiencies are compatible with survival can aid in predicting the magnitude of the risk associated with that particular inversion.
Another clue to the level of risk associated with a given inversion is the manner in which the inversion was ascertained. If a balanced inversion is ascertained fortuitously, for instance, during a prenatal chromosome study because of advanced maternal age, the risk associated with such an inversion is probably very low. On the other hand, an inversion that is ascertained through the birth of an infant with anomalies secondary to the presence of a recombinant chromosome is associated with a much higher risk since the important question of whether the recombinant offspring is viable has already been answered. Careful examination of the family history in both types of ascertainment can provide additional important information in assessing risk.
Gardner and Sutherland reviewed several studies that contain data about the risks associated with pericentric inversions and estimated the risk for an inversion heterozygote to have an abnormal child secondary to a recombinant chromosome [49]. This risk was estimated to be 5–10% in families ascertained through an abnormal child and approximately 1% for families ascertained for any other reason. For families segregating very small inversions, the risk of having a liveborn recombinant child may be close to zero. In cases of recurring inversions, additional information about the risks can be gained from studying the literature. In the case of the inversion (8)(p23q22) mentioned earlier, for example, enough recombinant offspring have been observed to derive an empiric risk of 6% for a heterozygote to have a liveborn recombinant child [87]. Large inversions with distal segments that have been seen in liveborn children as monosomies or trisomies may be associated with high risk regardless of their mode of ascertainment in a particular family.
Paracentric Inversions
The presence of paracentric inversions in the human population was only appreciated after the advent of chromosome banding, and they are still reported less frequently than pericentric inversions. Their incidence has been estimated at 0.09–0.49 per thousand [80]. While various cytogenetically visible recurrent paracentric inversions have been reported in a variety of chromosomes, recent data suggests that most do not represent multiple independent mutation events but are instead identical by decent [83]. One of the most frequently cited is the recurring inv(11)(q21q23), which is believed to have arisen as a single mutation in the Netherlands and is now observed in a large number of families in the Netherlands as well as in Canadian Hutterites [88, 89]. With the aid of fluorescence in situ hybridization (FISH) and other molecular techniques, a number of recurring submicroscopic inversions within the human genome are being identified.
Several studies have now demonstrated that some recurring submicroscopic inversions confer susceptibility to other rearrangements. For example, a submicroscopic inversion polymorphism that spans the same low-copy repeat sequences that mediate the recurring Williams syndrome deletion has been observed in the transmitting parent of some Williams syndrome parents [65–68]. The presence of a submicroscopic inversion polymorphism has also been associated with several other recurring microdeletions including Sotos, Angelman, the 15q13.3, 16p12.1, and 17q21.3 microdeletion syndromes, as well as several other types of disease-associated structural rearrangements. For example, submicroscopic inversions that span the olfactory receptor gene clusters at both 4p16 and 8p23, and mediate the recurring (4;8) translocation, have been seen in all nine of the transmitting parents examined [8, 90]. The same 8p inversion polymorphism associated with this (4;8) translocation has also been observed in each of the nine mothers who transmitted a recurring inverted duplicated 8p or supernumerary derivative 8p chromosome to their offspring [91]. Recombination between the homologous PRKX and PRKY genes located on the short arm of the X and Y chromosome, respectively, results in a translocation involving the material distal to the involved breakpoints and the formation of both XX males and XY females. This particular recombination event also appears to occur preferentially in association with a Yp inversion polymorphism [92].
The manner in which small paracentric inversion polymorphisms promote the formation of some chromosome rearrangements is not currently known in most cases. It has been demonstrated, however, that in the case of the recurring 15q13.3, 16p12.1, and 17q21.31 microdeletions the inversion produces an improved substrate for nonallelic homologous recombination (NAHR). In both cases, the inversion converts a pair of inverted LCRs into a pair of direct LCRs that are ideally suited to mediate the causative unequal exchange event [48, 69, 70].
Meiotic Behavior and Risk for Carriers of Paracentric Inversions
As with pericentric inversions, the classic solution to the problem of homologous pairing in paracentric inversions is the reverse loop. In this case, however, the centromeres are found in the segment distal to the inversion loop. On a theoretical basis, an odd number of crossovers within the inversion loop of a paracentric inversion should produce one dicentric and one acentric recombinant chromosome (Fig. 9.12). The dicentric recombinant is genetically unstable because each of the two centromeres could potentially orient toward opposite poles of the dividing cell. The material between the two centromeres would remain stretched between the poles of the two reorganizing daughter nuclei or break. Thus, with each cell division, the dicentric recombinant chromosome has a new opportunity to contribute a different and possibly lethal genetic imbalance to a new generation of cells. The acentric fragment, on the other hand, has no ability to attach to a spindle since it lacks a centromere. Consequently, at cell division, it can be passively included in the daughter nuclei or be lost. Dicentric and acentric recombinant chromosomes are almost always lethal and are rarely found in liveborns (see sections “Acentric Chromosomes” and “Dicentric Chromosomes,” later).


Fig. 9.12
The type of recombinant chromosome produced depends on which mechanism of chromosome exchange occurs within the paracentric inversion loop. A classic crossover within the inversion loop results in the formation of an acentric and a dicentric recombinant chromosome (top), whereas a U-type exchange produces only monocentric chromosomes (bottom)
Although dicentric and acentric recombinants are very rarely seen, there have been several reports of monocentric recombinants among the children of paracentric inversion carriers. Pettenati et al., for example, identified 17 recombinant chromosomes among 446 inversions [80]. While two of these recombinant chromosomes were dicentric, each of the remaining 15 was monocentric with duplications and/or deletions. A variety of mechanisms have now been proposed for the formation of these abnormal monocentric chromosomes, including breakage of dicentric recombinants, unequal crossing-over, and abnormal U-loop exchanges similar to the one diagrammed in Fig. 9.12. All of these mechanisms involve abnormal processes of one type or another.
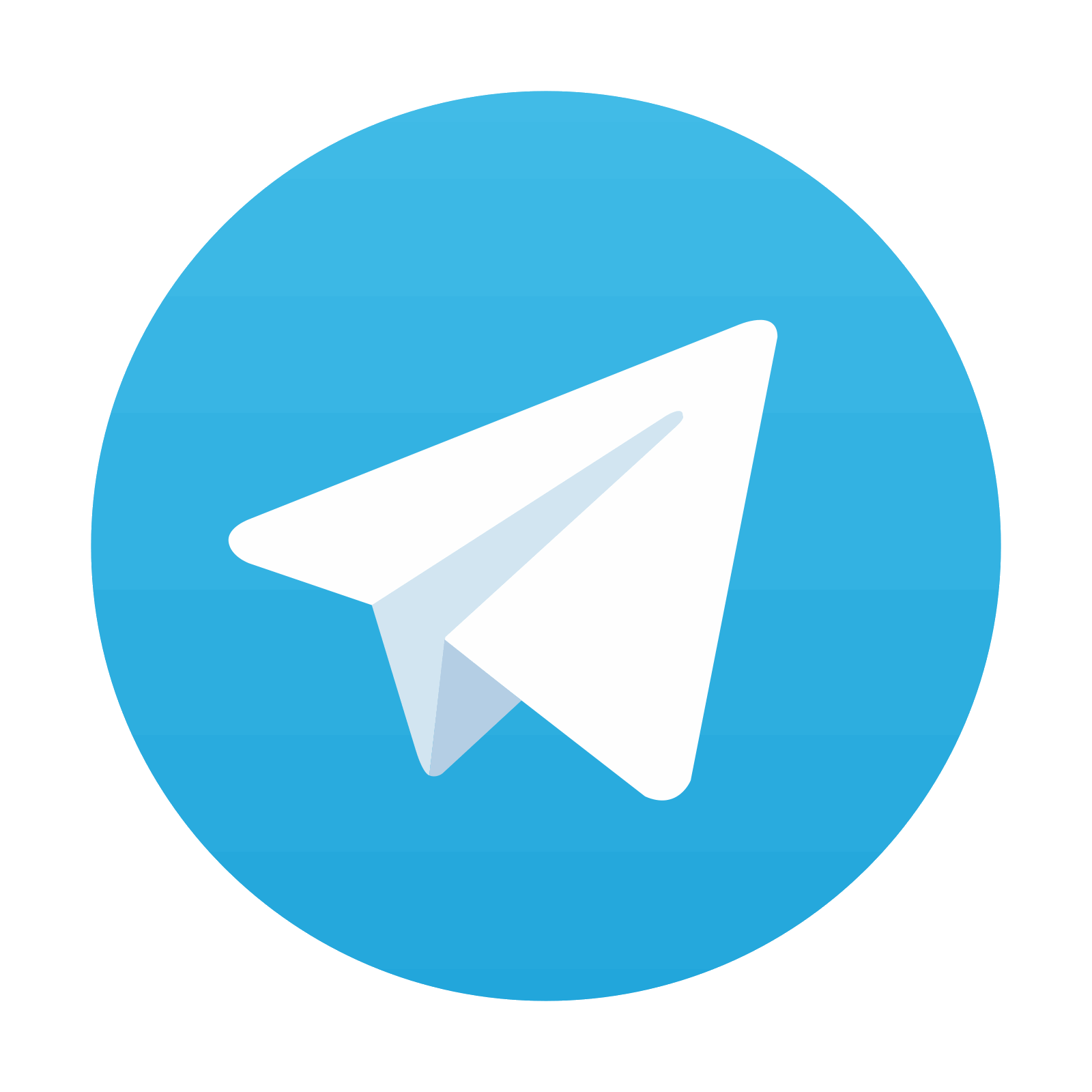
Stay updated, free articles. Join our Telegram channel
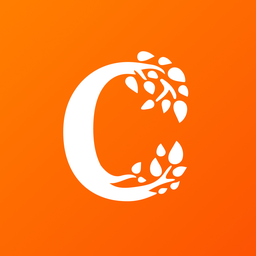
Full access? Get Clinical Tree
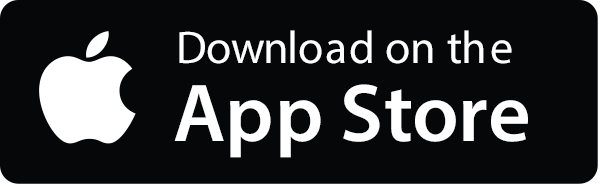
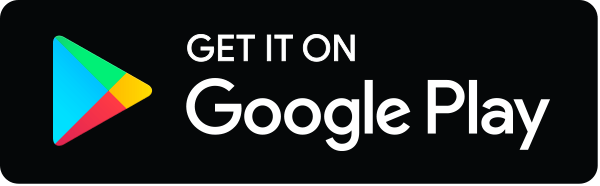