Chapter 3
The Cellular Environment
Fluids and Electrolytes, Acids and Bases
Alexa K. Doig and Sue E. Huether
Distribution of Body Fluids
The fluids of the body are distributed among functional compartments, or spaces, and provide a transport medium for cellular and tissue function. Water moves freely among body compartments and is distributed by osmotic and hydrostatic forces. Two thirds of the body’s water is intracellular fluid (ICF) and one third is in the extracellular fluid (ECF) compartments. The two main ECF compartments are the interstitial fluid and the intravascular fluid, the latter being the blood plasma. Other ECF compartments include the lymph and the transcellular fluids, such as the saliva, intestinal, biliary, hepatic, pancreatic, and cerebrospinal fluids; sweat; urine; and pleural, synovial, peritoneal, pericardial, and intraocular fluids (Table 3-1).
TABLE 3-1
APPROXIMATE CONCENTRATIONS OF ELECTROLYTES IN TRANSCELLULAR FLUIDS
FLUID | Na+ (mEq/L) | K+ (mEq/L) | Cl− (mEq/L) | ![]() |
Saliva | 33 | 20 | 34 | 40 |
Gastric juice∗ | 60 | 9 | 84 | 0 |
Bile | 149 | 5 | 101 | 45 |
Pancreatic juice | 141 | 5 | 77 | 92 |
Ileal fluid | 129 | 11 | 116 | 29 |
Cecal fluid | 80 | 21 | 48 | 22 |
Cerebrospinal fluid | 141 | 3 | 127 | 23 |
Sweat | 45 | 5 | 58 | 0 |
∗The Cl− concentration exceeds the Na+, K+ concentration by 15 mEq/L in gastric juice. This largely represents the secretions of hydrochloric acid by parietal cells.
The sum of fluids within all compartments constitutes the total body water (TBW) (Table 3-2). The volume of TBW is usually expressed as a percentage of body weight in kilograms. The standard value for TBW is 60% of the weight of a 70-kg adult male, which is equivalent to 42 L of fluid (Table 3-3). The rest of the body weight is composed of fat and fat-free solids, particularly bone.
TABLE 3-2
PERCENTAGE OF BODY WEIGHT | VOLUME (L) | |
Intracellular fluid (ICF) | 40 | 28 |
Extracellular fluid (ECF) | 20 | 14 |
(15) | (11) | |
(5) | (3) | |
Total body water (TBW) | 60 | 42 |
TABLE 3-3
TOTAL BODY WATER∗ IN RELATION TO BODY WEIGHT
BODY BUILD | TBW (%) ADULT MALE | TBW (%) ADULT FEMALE | TBW (%) INFANT |
Normal | 60 | 50 | 70 |
Lean | 70 | 60 | 80 |
Obese | 50 | 42 | 60 |
∗NOTE: TBW (total body water) is a percentage of body weight.
Although daily fluid intake may fluctuate widely, the body regulates water volume within a relatively narrow range. The primary sources of body water are drinking of fluids, ingestion of water in food, and derivation of water from oxidative metabolism. Normally, the largest amounts of water are lost through renal excretion. Lesser amounts are eliminated through the stool and through vaporization from the skin and lungs (insensible water loss) (Table 3-4).
TABLE 3-4
NORMAL WATER GAINS AND LOSSES (70-kg MAN)
DAILY INTAKE (ml) | DAILY OUTPUT (ml) | ||
Drinking ≈60% | 1400-1800 | Urine ≈60% | 1400-1800 |
Water in food ≈30% | 700-1000 | Stool ≈2% | 100 |
Water of oxidation ≈10% | 300-400 | Skin ≈10% | 300-500 |
Lungs ≈28% | 600-800 | ||
total | 2400-3200 | 2400-3200 |
Aging and Distribution of Body Fluids
The distribution and amount of TBW change with age (see Table 3-3). In newborn infants, TBW is about 75% to 80% of body weight because infants store less fat. In the immediate postnatal period, a physiologic loss of body water occurs, equivalent to about 5% of body weight as the infant adjusts to a new environment. Infants are particularly susceptible to significant changes in TBW because of their high metabolic rate and potential for evaporative fluid loss attributable to their greater body surface area in proportion to total body size. Loss of fluids from diarrhea can represent a significant proportion of body weight in infants. Renal mechanisms that regulate fluid and electrolyte conservation may not be mature enough to counter the losses, so dehydration can develop rapidly.
With increasing age the percentage of TBW declines further still. The decrease is caused in part by an increased amount of fat and a decreased amount of muscle and by a reduced ability to regulate sodium and water balance. With older age the kidneys becomes less efficient at conserving sodium and therefore have difficulty concentrating the urine. Insensible water loss through the skin may increase and thirst perception may be impaired. The normal reduction of TBW in older adults becomes clinically important when the body is under stress, such as development of fever or dehydration; loss of body fluids at such times can be severe and life threatening.1
Water Movement Between ICF and ECF
The movement of water between ICF and ECF compartments is primarily a function of osmotic forces. (Osmosis and other mechanisms of passive transport are discussed in Chapter 1.) Water moves freely by diffusion through the lipid bilayer cell membrane and through aquaporins, a family of water channel proteins that provide permeability to water.2 The osmolality (number of osmoles of solute per kilogram of fluid [Osm/kg]) of TBW is normally at equilibrium. Sodium is responsible for the osmotic balance of the ECF space. Potassium maintains the osmotic balance of the ICF space. The osmotic force of ICF proteins and other nondiffusible substances is balanced by the active transport of ions out of the cell. Water crosses cell membranes freely so the osmolality of TBW is normally at equilibrium. Normally the ICF is not subject to rapid changes in osmolality but when ECF osmolality changes, water moves from one compartment to another until osmotic equilibrium is reestablished (see Figure 3-6, p. 110).
Water Movement Between Plasma and Interstitial Fluid
1. Capillary hydrostatic pressure (blood pressure) facilitates the outward movement of water from the capillary to the interstitial space.
2. Capillary (plasma) oncotic pressure osmotically attracts water from the interstitial space back into the capillary.
3. Interstitial hydrostatic pressure facilitates the inward movement of water from the interstitial space into the capillary.
4. Interstitial oncotic pressure osmotically attracts water from the capillary into the interstitial space.



As the plasma flows from the arterial to the venous end of the capillary, the force of hydrostatic pressure facilitates the movement of water across the capillary membrane. Oncotic pressure remains fairly constant because plasma proteins normally do not cross the capillary membrane. At the arterial end of the capillary, hydrostatic pressure is greater than capillary oncotic pressure and water filters into the interstitial space. Because of oncotic forces, some water moves back into the capillary, but the net effect is loss of water from the capillary. This loss of water from the plasma decreases the hydrostatic pressure within the capillary; thus at the venous end of the capillary, oncotic pressure exceeds hydrostatic pressure. Fluids then are attracted back into the circulation, balancing the movement of fluids between the plasma and the interstitial space. The overall effect is filtration at the arterial end and reabsorption at the venous end (Figure 3-1). Interstitial hydrostatic pressure promotes the movement of about 10% of the interstitial fluid along with small amounts of protein into the lymphatics, which eventually returns to the circulation.
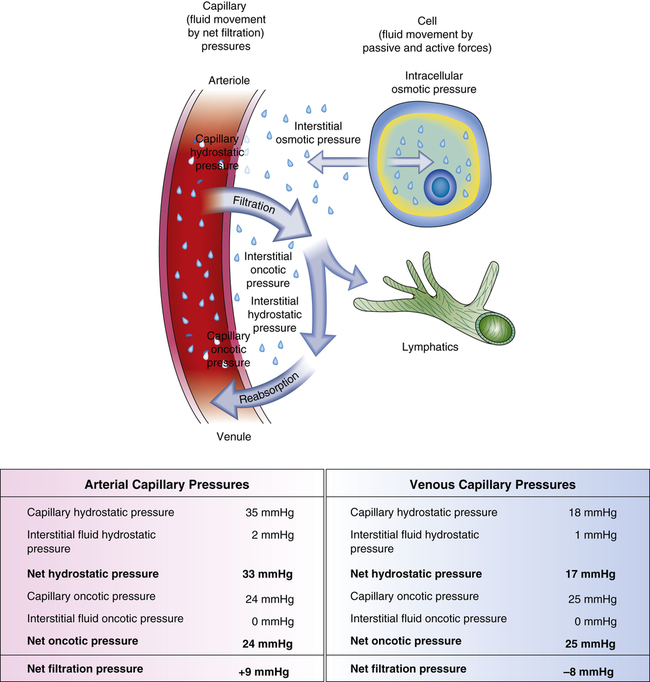
Water, electrolytes, and small molecules exchange freely between the vascular compartment and the interstitial space at the site of capillaries and small venules. The rate and amount of exchange are driven by the physical forces of hydrostatic and oncotic pressures and the permeability and surface area of the capillary membranes. The two opposing hydrostatic pressures are capillary hydrostatic pressure and interstitial hydrostatic pressure. The two opposing oncotic pressures are capillary oncotic pressure and interstitial oncotic pressure. The forces that favor filtration from the capillary are capillary hydrostatic pressure and interstitial oncotic pressure, and the forces that oppose filtration are capillary oncotic pressure and interstitial hydrostatic pressure. The sum of their effects is known as net filtration pressure (NFP). In the example of normal exchange illustrated here, a small amount of fluid moves to the lymph vessels, which accounts for the net filtration difference between the arterial and venous ends of the capillary.
Alterations in Water Movement
Edema
1. Increased capillary hydrostatic pressure
2. Decreased plasma oncotic pressure
3. Increased capillary membrane permeability
4. Lymphatic obstruction (Figure 3-2)
Pathophysiology
Increased capillary permeability is usually associated with inflammation and the immune response. (Immunity is discussed in Chapters 7, 8, and 9; inflammation is discussed in Chapters 7 and 9.) These responses are often the result of trauma such as burns or crushing injuries, neoplastic disease, allergic reactions, and infection. Excess amounts of fluid escape from the plasma to the interstitial space and produce edema. This type of edema is often very severe because of loss of proteins from the vascular space, which decreases capillary oncotic pressure and increases interstitial oncotic pressure.
Lymphatic obstruction occurs when the lymphatic channels are blocked because of infection or tumor. Proteins and fluids are not reabsorbed and accumulate in the interstitial space, causing lymphedema. Lymphedema of the arm or leg also can occur after surgical removal of axillary or femoral lymph nodes, respectively, for treatment of cancer.3
Clinical Manifestations
Generalized edema is manifested by a more uniform distribution of fluid in interstitial spaces throughout the body. Dependent edema, in which fluid accumulates in gravity-dependent areas of the body, might appear in the feet and legs when standing and in the sacral area and buttocks when supine. Dependent edema can be identified by using the fingers to press away edematous fluid in tissues overlying bony prominences. A pit will be left in the skin; hence the term pitting edema (Figure 3-3).
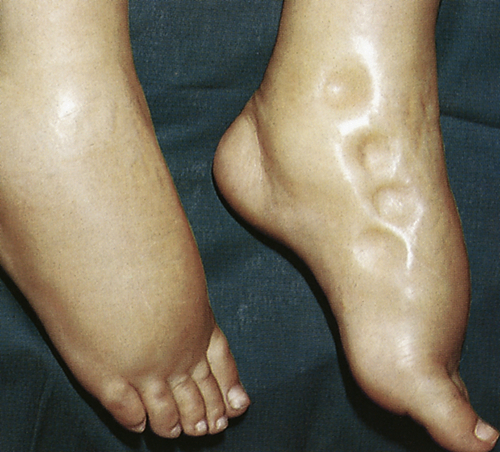
Edema is usually associated with swelling and puffiness, tight-fitting clothes and shoes, and limited movement of the affected area. Weight gain can be significant. The accumulation of fluid increases the distance required for nutrients, oxygen, and wastes to move between capillaries and cells in the tissues. Increased tissue pressure also may diminish capillary blood flow, leading to ischemia. Therefore, wounds heal more slowly and formation of pressure sores increases (see Chapter 46).
As edematous fluid accumulates, it is trapped in a “third space” (i.e., the interstitial space) and dehydration can develop as a result of this sequestering of fluid. Such sequestration occurs with severe burns, in which large amounts of vascular fluid are lost to the interstitial spaces, reducing plasma volume and causing shock (see Chapter 48).
Sodium, Chloride, and Water Balance
Sodium and Chloride Balance
Sodium accounts for 90% of the ECF cations (positively charged ions). The distribution of electrolytes in body compartments is summarized in Table 3-5 and the concentration of electrolytes is summarized in Table 3-1. As the most abundant ECF cation, along with its constituent anions (negatively charged ions) chloride and bicarbonate, sodium regulates extracellular osmotic forces and therefore regulates water balance. Sodium is important in other body functions, including maintenance of neuromuscular irritability for conduction of nerve impulses (in conjunction with potassium and calcium), regulation of acid-base balance (through sodium bicarbonate and sodium phosphate), participation in cellular chemical reactions, and transport of substances across the cellular membrane (see Chapter 1).
TABLE 3-5
DISTRIBUTION OF ELECTROLYTES IN BODY COMPARTMENTS
EXTRACELLULAR FLUID (mEq/L) | INTRACELLULAR FLUID (mEq/L) | |
Cations | ||
142 | 10 | |
5 | 156 | |
5 | 4 | |
2 | 26 | |
total | 154 | 196 |
Anions | ||
24 | 12 | |
104 | 4 | |
2 | 40-95 | |
16 | 54 | |
8 | 31-86 | |
total | _____ 154 | ____________ 196 (average) |
Hormonal regulation of sodium balance is mediated by aldosterone, a mineralocorticoid (steroid) synthesized and secreted from the adrenal cortex as the end product of the renin-angiotensin-aldosterone system (Figure 3-4) (also see Chapters 21 and 37). When circulating blood pressure and renal blood flow, or serum sodium concentrations, are reduced, renin, an enzyme secreted by the juxtaglomerular cells of the kidney, is released. Renin stimulates the formation of angiotensin I, an inactive polypeptide. Angiotensin-converting enzyme (ACE) in pulmonary vessels converts angiotensin I to angiotensin II. Angiotensin II has two major functions: it causes vasoconstriction, which elevates systemic blood pressure, and it stimulates the secretion of aldosterone. Aldosterone promotes sodium and water reabsorption by the proximal tubules of the kidneys, thus conserving sodium, blood volume, and blood pressure. Aldosterone also stimulates secretion (and therefore excretion) of potassium by the distal tubule of the kidney, reducing potassium concentrations in the ECF. The restoration of sodium levels, blood volume, and renal perfusion then inhibits further release of renin.
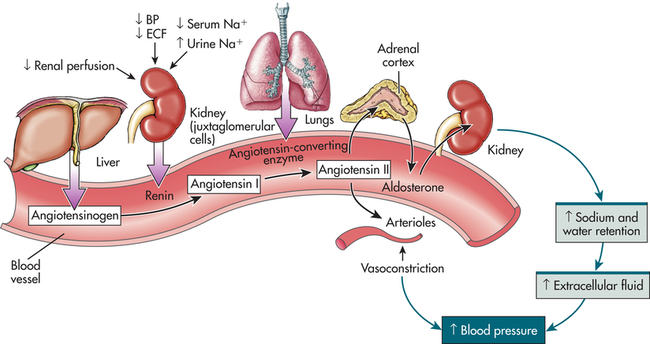
BP, Blood pressure; ECF, extracellular fluid; Na, sodium. (From Lewis et al: Medical-surgical nursing: assessment and management of clinical problems, ed 8, St. Louis, 2011, Mosby.)
Natriuretic peptides are hormones that include atrial natriuretic peptide (ANP) produced by myoendocrine atrial cells, brain natriuretic peptide (BNP—named brain since it was first discovered in porcine brain) produced by myoendocrine ventricular cells, and urodilatin (an ANP analog) synthesized within the kidney. ANP and BNP are released when there is an increase in transmural atrial pressure caused by increased intra-atrial volume as may occur with heart failure.4 ANP and BNP increase sodium and water excretion by the kidneys, which lowers blood volume and pressure. Urodilatin is released from distal tubular kidney cells when there is increased arterial pressure and increased renal blood flow. These hormones are natural antagonists to the renin-angiotensin-aldosterone system. The restoration of lower atrial pressure then inhibits further release of ANP and BNP.
Water Balance
Water balance also is directly regulated by antidiuretic hormone (arginine-vasopressin), which is secreted when plasma osmolality increases or circulating blood volume decreases and blood pressure drops (Figure 3-5). Increased plasma osmolality occurs with a water deficit or sodium excess in relation to water. The increased osmolality stimulates hypothalamic osmoreceptors. In addition to causing thirst, the stimulated osmoreceptors signal the posterior pituitary to release ADH. The action of ADH is to increase the permeability of renal tubular cells to water, increasing water reabsorption and promoting the restoration of plasma volume and blood pressure. Urine concentration increases, and the reabsorbed water decreases plasma osmolality, returning it toward normal. Like most hormones, ADH is regulated by a feedback mechanism. The restoration of plasma osmolality, blood volume, and blood pressure then inhibits ADH secretion.
With fluid loss (dehydration) (e.g., from vomiting, diarrhea, or excessive sweating), a decrease in blood volume and blood pressure often occurs. Baroreceptors (volume/pressure sensitive receptors) (stretch receptors that are sensitive to changes in arterial volume and pressure) also stimulate the release of ADH. Baroreceptors are located in the right and left atria and large veins, and in the aorta, pulmonary arteries, and carotid sinus. When arterial and atrial pressure drops baroreceptors signal the hypothalamus to release ADH. The reabsorption of water mediated by ADH then promotes the restoration of plasma volume and blood pressure (see Figure 3-5). ADH also stimulates arterial vasoconstriction.
Alterations in Sodium, Chloride, and Water Balance
Alterations in sodium and water balance are closely related. Water imbalances may develop because of changes in osmotic gradients caused by gain or loss of salt. Likewise, sodium imbalances occur with alterations in body water volume. Generally the alterations can be classified as changes in tonicity, or the change in concentration of electrolytes in relation to water (see Chapter 1). Alterations can therefore be classified as isotonic, hypertonic, or hypotonic (Table 3-6 and Figure 3-6).
TABLE 3-6
TONICITY | MECHANISM |
Isotonic (isoosmolar) imbalance | Gain or loss of extracellular fluid (ECF) resulting in a concentration equivalent to a 0.9% sodium chloride (salt) solution (normal saline); no shrinking or swelling of cells |
Hypertonic (hyperosmolar) imbalance | Imbalance that results in an ECF concentration >0.9% salt solution; that is, water loss or solute gain; cells shrink in a hypertonic fluid |
Hypotonic (hypoosmolar) imbalance | Imbalance that results in an ECF <0.9% salt solution; that is, water gain or solute loss; cells swell in a hypotonic fluid |
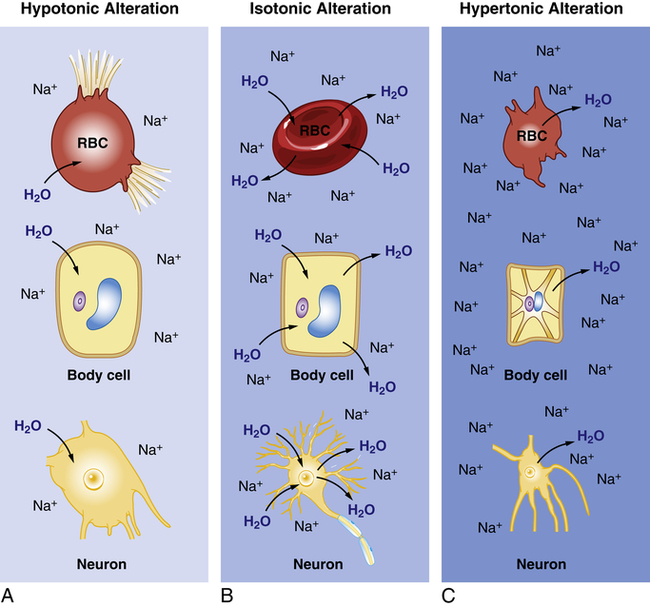
A, Hypotonic Alteration: Decrease in ECF sodium (Na) concentration (hyponatremia) results in ICF osmotic attraction of water with swelling and potential busting of cells. B, Isotonic Alteration: Normal concentration of sodium in the ECF and no change in shifts of fluid in or out of cells. C, Hypertonic Alteration: An increase in ECF sodium concentration (hypernatremia) results in osmotic attraction of water out of cells with cell shrinkage. RBC, Red blood cell.
Isotonic Alterations
The term isotonic refers to a solution that has the same concentration of solutes as the plasma. Isotonic alterations occur when changes in TBW are accompanied by proportional changes in the amounts of electrolytes and water. For example, if an individual loses pure plasma or ECF, fluid volume is depleted but the number and type of electrolytes (e.g., sodium) and the osmolality remain within a normal range. Excessive amounts of isotonic body fluids can result from excessive administration of intravenous normal saline (0.9% NaCl) or oversecretion of aldosterone with renal retention of both sodium and water. Isotonic fluid loss results in hypovolemia. Causes include hemorrhage, severe wound drainage, and excessive diaphoresis (sweating). Loss of extracellular volume results in weight loss, dryness of skin and mucous membranes, decreased urine output, increased hematocrit value, and symptoms of hypovolemia. Indicators of hypovolemia include a rapid heart rate and flattened neck veins, and can present with a normal or decreased blood pressure. In severe states, hypovolemic shock (severe hypotension) can occur (see Chapter 48).
Hypertonic Alterations
Hypertonic fluid alterations develop when the osmolality of the ECF is elevated above normal (greater than 294 mOsm). The most common causes are an increased concentration of ECF sodium (hypernatremia) or a deficit of ECF free water. In both instances the hypertonicity of the ECF attracts water from the intracellular space, causing ICF dehydration. A primary increase in the amount of ECF sodium causes an osmotic attraction of water and symptoms of hypervolemia. In contrast, a hypertonic state caused primarily by free water loss leads to hypovolemia (Table 3-7).
TABLE 3-7
CAUSES AND CONSEQUENCES OF HYPERTONIC IMBALANCES
CAUSATIVE FACTOR | MECHANISM | ECF EFFECTS | ICF EFFECTS |
Increased sodium (hypernatremia) | Excessive hypertonic salt solutions Intravenous hypertonic sodium Saline-induced abortions Selected infant formulas Hyperaldosteronism Cushing syndrome | Hypervolemia Weight gain Bounding pulse Increased blood pressure Edema Venous distention Neuromuscular symptoms Muscle weakness Seizures | Intracellular dehydration Thirst Fever Decreased urine output Shrinkage of brain cells Confusion Coma Cerebral hemorrhage |
Water deficit | Water deprivation Confusion or coma Inability to communicate Loss of thirst Water loss Watery diarrhea Diabetes insipidus Excessive diuresis Excessive diaphoresis | Hypovolemia Weight loss Weak pulses Postural hypotension Tachycardia | Intracellular dehydration See above |
Other factors | Hyperglycemia | Initial dilutional hyponatremia Polyuria Polydipsia Weight loss Hypovolemia Late hypernatremia | Intracellular dehydration See above |
Hypernatremia
Pathophysiology
Hypernatremia occurs when serum sodium levels exceed 147 mEq/L. Increased serum sodium concentration may be caused by loss of water (most common) or an acute gain in sodium. With water loss, both ICF dehydration and ECF dehydration occur. Hyperosmolality is a common result of hypernatremia. Because sodium is largely in the ECF, increases in the concentration of sodium cause intracellular dehydration and hypervolemia (see Table 3-7 and Figure 3-6, C).
Increased sodium retention occurs because of (1) inappropriate administration of hypertonic saline solution (e.g., as sodium bicarbonate for treatment of acidosis during cardiac arrest); (2) oversecretion of aldosterone (as in primary hyperaldosteronism), where sodium reabsorption exceeds water reabsorption; or (3) Cushing syndrome (caused by excess secretion of adrenocorticotropic hormone [ACTH], which also causes increased secretion of aldosterone). High amounts of dietary sodium rarely cause hypernatremia in a healthy individual because the sodium is eliminated by the kidneys. However, increased amounts of dietary sodium (greater than 5 grams per day) is associated with cardiovascular disease (see Chapter 32).
Evaluation and Treatment
The serum sodium level is usually more than 147 mEq/L. If there is water loss, urine specific gravity will be greater than 1.030 and the levels of hematocrit and plasma proteins will be elevated. The treatment of hypernatremia is to give an isotonic salt-free fluid (5% dextrose in water) until the serum sodium level returns to normal.5 Fluid replacement must be given slowly to prevent cerebral edema and serum sodium levels need to be closely monitored. Hypervolemia and hypovolemia require treatment of the underlying clinical condition.
Hyperchloremia
Because chloride follows sodium, hyperchloremia (elevation of serum chloride concentration greater than 105 mEq/L) often accompanies hypernatremia, as well as plasma bicarbonate deficits, as in hyperchloremic metabolic acidosis (see p. 126). There are no specific symptoms or treatment for chloride excess and treatment is related to management of the underlying disorder.
Water Deficit
Pathophysiology
Dehydration refers to water deficit, but also is commonly used to indicate both sodium and water loss (isotonic or isoosmolar dehydration). Pure water deficits (hyperosmolar or hypertonic dehydration) are rare because most people have access to water. Individuals who are comatose or paralyzed continue insensible water losses through the skin and lungs with a minimal obligatory formation of urine. Hyperventilation caused by fever also may precipitate water deficit. The most common cause of water loss is increased renal clearance of free water as a result of impaired tubular function or inability to concentrate the urine, as with diabetes insipidus (decreased or absent ADH) (see Table 3-7 and Chapter 22).
Hypotonic Alterations
Hypotonic fluid imbalances occur when the osmolality of the ECF is less than normal (less than 280 mOsm). The most common causes are sodium deficit (hyponatremia) or free water excess (water intoxication). Both causes lead to an intracellular overhydration (cellular edema) and cell swelling when water moves into the cell, where the osmotic pressure is greater (see Figure 3-6, A). Cerebral and pulmonary edema occur in conjunction with these fluid shifts.6 With hyponatremia, the plasma volume then decreases, leading to symptoms of hypovolemia. With free water excess, the ECF volume is elevated, causing symptoms of hypervolemia (Table 3-8).
TABLE 3-8
CAUSES AND CONSEQUENCES OF HYPOTONIC IMBALANCES
CAUSATIVE FACTOR | MECHANISM | ECF EFFECTS | ICF EFFECTS |
Decreased sodium (hyponatremia) | Inadequate intake Hypoaldosteronism Excessive diuretic therapy Furosemide Ethacrynic acid Thiazides | Extracellular volume contraction and hypovolemia (but may not if there is water excess) | Increased intracellular water; edema Brain cell swelling, irritability, depression, confusion Systemic cellular edema, including weakness, anorexia, nausea, and diarrhea |
Water excess | Excessive pure water intake Excessive administration of hypotonic intravenous solutions Drinking water to replace isotonic fluid losses Tap water enemas Psychogenic polydipsia Renal water retention Syndrome of inappropriate secretion of antidiuretic hormone (SIADH) | Extracellular volume expands with hypervolemia (but may not if fluid is trapped in intracellular space) | Edema (see above) |
Other factors | Isotonic dehydration treated with intravenous D5W; glucose in D5W solution is metabolized to water, contributing to hyponatremia Nephrotic syndrome Cirrhosis Cardiac failure | Hypervolemia or hypovolemia | Edema (see above) |
D5W, Dextrose 5% in water; ECF, extracellular fluid; ICF, intracellular fluid.
Hyponatremia
Pathophysiology
Hyponatremia develops when the serum sodium concentration decreases to less than 135 mEq/L. It is the most common electrolyte disorder in hospitalized individuals.7 Hyponatremia may be caused by sodium loss, inadequate sodium intake, or dilution of the body’s sodium level. Clinical syndromes that may cause hyponatremia include syndrome of inappropriate secretion of antidiuretic hormone (SIADH, excess ADH) or failure of the distal tubules to reabsorb sodium. Sodium deficits usually cause hypoosmolality and water moves into cells, resulting in cell swelling (see Figure 3-6, A, and What’s New? Hyponatremia and Hospitalization).
Pure sodium deficits are usually caused by diuretics8 and extrarenal losses such as vomiting, diarrhea, gastrointestinal suctioning, or burns. Inadequate intake of dietary sodium is rare but can occur in individuals consuming low-sodium diets, particularly among those taking diuretics. Dilutional hyponatremias occur when there is an excess of TBW in relation to total body sodium or a shift of water from the ICF to the ECF space (e.g., administration of mannitol). Replacement of fluid loss with intravenous 5% dextrose in water also can cause a dilutional hyponatremia because once the glucose is metabolized, a hypotonic solution remains with a diluting effect. Use of excess hypotonic saline (e.g., 0.45% NaCl) may also result in dilution. In addition, excessive sweating may stimulate thirst and intake of large amounts of water, which dilute sodium; this condition may be associated with endurance exercise in which there is only pure water replacement.
Clinical Manifestations
Cellular swelling and deficits of intracellular sodium alter the ability of cells to depolarize and repolarize normally (see Chapter 1). Neurologic changes characteristic of hyponatremia include lethargy, headache, confusion, apprehension, seizures, and coma. Pure sodium losses may be accompanied by loss of ECF, causing hypovolemia with symptoms of hypotension, tachycardia, and decreased urine output. Dilutional hyponatremia is accompanied by weight gain, edema, ascites, and jugular vein distention. Cerebral edema can be a life-threatening complication of hypervolemic hyponatremia caused by increases in intracranial pressure.
Evaluation and Treatment
Treatment of hyponatremia is related to the contributing disorder. Losses of sodium and water volume are calculated from the clinical evaluation, and appropriate solutions then are selected for replacement. Restriction of water intake is required in most cases of dilutional hyponatremia because body sodium levels may be normal or increased even though serum concentrations are low. Hypertonic saline solutions are used cautiously with severe hyponatremia or the presence of symptoms such as seizures.9
Hypochloremia
Hypochloremia, a low level of serum chloride (less than 97 mEq/L), usually occurs with hyponatremia or an elevated bicarbonate concentration, as in metabolic alkalosis (see p. 128). Sodium deficit related to restricted intake, use of diuretics, and vomiting are accompanied by chloride deficiency. Cystic fibrosis is a genetic disease characterized by hypochloremia (see Chapters 36 and 42). In all cases, treatment of the underlying cause is required.
Water Excess
Pathophysiology
When the body is functioning normally, it is almost impossible to produce an excess of TBW since water balance is regulated by the kidneys. However, some individuals with psychogenic disorders develop water intoxication from compulsive water drinking. Intensive exercise with replacement of large volumes of electrolyte-free water also causes overhydration. Acute renal failure, severe congestive heart failure, and cirrhosis are clinical conditions that can precipitate water excess. Decreased urine formation from renal disease or decreased renal blood flow contributes to water excess. The overall effect is dilution of the ECF with the movement of water to the intracellular space by osmosis. The syndrome of inappropriate secretion of ADH (SIADH), also known as vasopressin dysregulation, enhances water retention because ADH levels are elevated (see Chapter 22, p. 718). Water excess is usually accompanied by hyponatremia.
Alterations in Potassium, Calcium, Phosphate, and Magnesium Balance
Potassium
Potassium (K+) is the major intracellular electrolyte and is found in most body fluids (see Table 3-5, p. 108). The ICF concentration of K+ is 150 to 160 mEq/L; the ECF concentration is 3.5 to 5.0 mEq/L. Total body potassium content is about 4000 mEq, with most of it located in the cells. Daily dietary intake of potassium is 40 to 150 mEq/day, with an average of 1.5 mEq/kg body weight.
The difference in the K+ intracellular to extracellular concentration is maintained by a sodium-potassium active transport system (Na+-K+-ATPase pump). The ratio of ICF K+ concentration to ECF K+ concentration is the major determinant of the resting membrane potential, which is necessary for the transmission and conduction of nervous impulses, maintenance of normal cardiac rhythms, and contraction of skeletal and smooth muscles (see Figure 1-35, p. 35). The constant diffusion of positively charged K+ out of the cell (i.e., down its concentration gradient) makes the interior of cells electronegative in relation to the ECF. Changes in the ratio of ICF to ECF potassium are responsible for many of the symptoms associated with K+ imbalance.
The kidney provides the most efficient regulation of K+ level balance over time. The amount of K+ excreted varies in proportion to the dietary intake (40 to 120 mEq/day). Potassium is freely filtered by the renal glomerulus, and 90% is reabsorbed by the proximal tubule and loop of Henle. Principal cells in the collecting duct secrete K+ and intercalated cells in the collecting duct reabsorb K+. Dietary K+ intake, aldosterone level, and distal tubule urine flow determine the amount of K+ excreted from the body. Unlike sodium, the renal mechanism for conserving K+ is weak, even when total body K+ stores are depleted. The gut also may sense the amount of K+ ingested and stimulate renal K+ excretion.10 However, a low K+ intake also suppresses renal K+ excretion.
Renal regulation of potassium level includes:
1. The concentration gradient for K+ at the distal tubule and collecting duct
2. The distal tubule flow rate and distal tubule sodium delivery
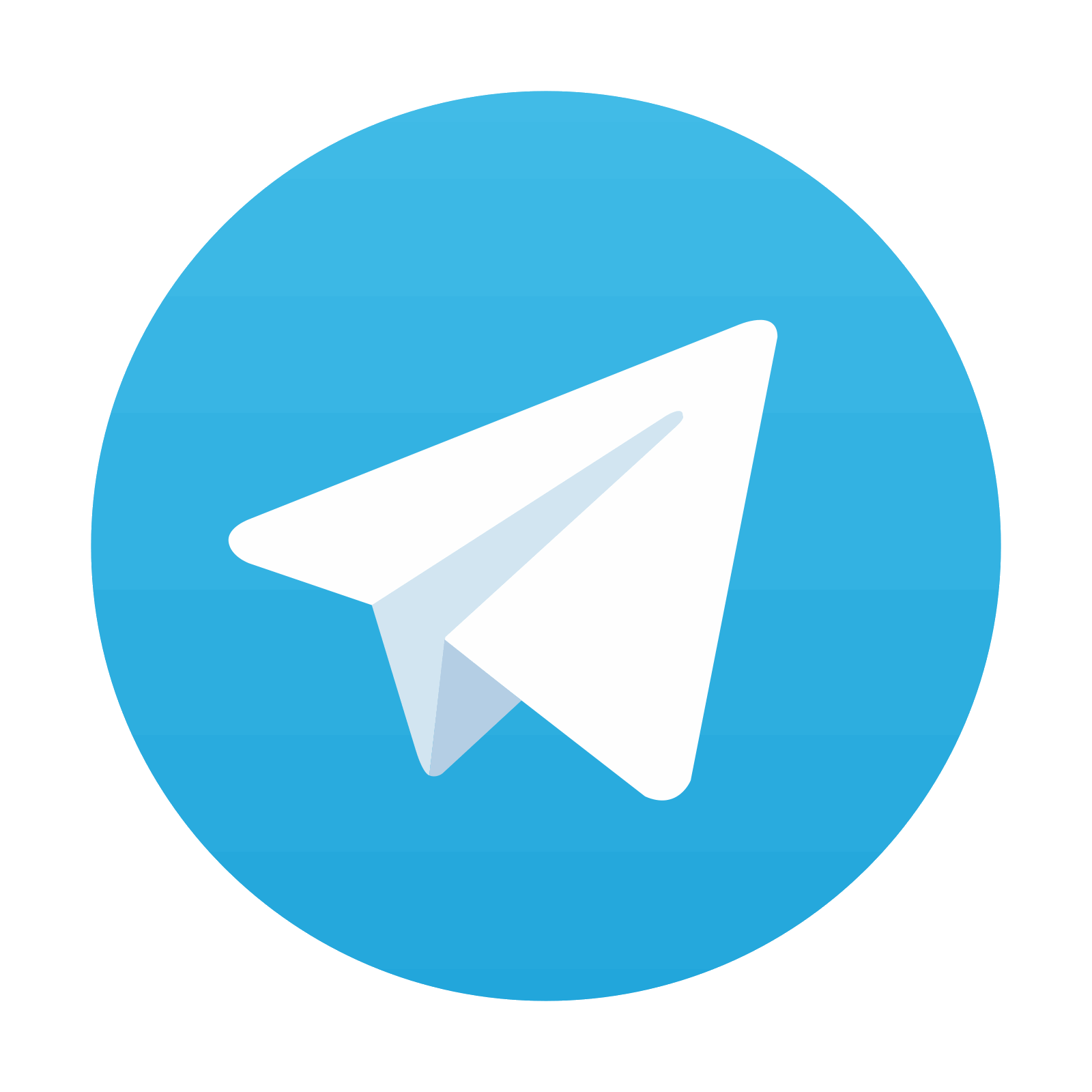
Stay updated, free articles. Join our Telegram channel
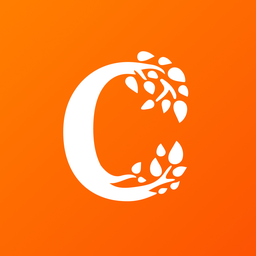
Full access? Get Clinical Tree
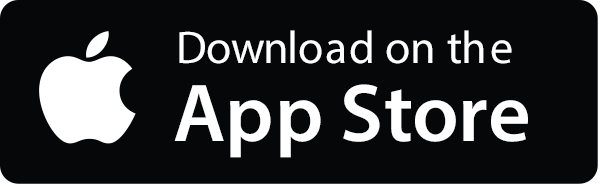
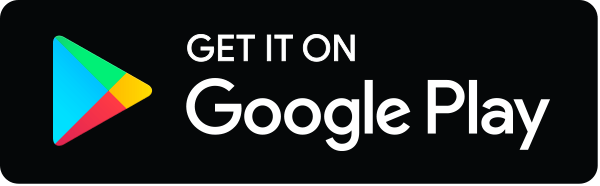