Chapter 5
The cardiovascular system
5.1 Blood vessels 83
5.4 Left ventricular valves 88
5.5 Heart valves and sounds 89
5.7 Conduction of heart impulses 90
5.8 Events represented by the ECG 94
5.9 Pulse points 99
5.10 Pulmonary circulation 100
5.11 Hepatic portal circulation 110
5.12 Fetal circulation 115
5.13 The effects of atheroma on blood flow 121
• the heart, whose pumping action ensures constant circulation of the blood
• the blood vessels, which form a lengthy network through which the blood flows.
The lymphatic system is closely connected, both structurally and functionally, with the cardiovascular system and is discussed in Chapter 6.
The heart pumps blood into two anatomically separate systems of blood vessels (Fig. 5.1):
The right side of the heart pumps blood to the lungs (the pulmonary circulation) where gas exchange occurs, i.e. the blood collects oxygen from the airsacs and excess carbon dioxide diffuses into the airsacs for exhalation. The left side of the heart pumps blood into the systemic circulation, which supplies the rest of the body. Here, tissue wastes are passed into the blood for excretion, and body cells extract nutrients and oxygen.
The cardiovascular system ensures a continuous flow of blood to all body cells, and its function is subject to continual physiological adjustments to maintain an adequate blood supply. Should the supply of oxygen and nutrients to body cells become inadequate, tissue damage occurs and cell death may follow.
Cardiovascular function normally declines with age, which is discussed on p. 117. Disease of the cardiovascular system is likely to have significant consequences, not only for the heart and blood vessels, but also for other body systems, which is discussed from page 118.
Blood vessels
Blood vessels vary in structure, size and function, and there are several types: arteries, arterioles, capillaries, venules and veins (Fig. 5.2). 5.1
Arteries and arterioles
These blood vessels transport blood away from the heart. They vary considerably in size and their walls consist of three layers of tissue (Fig. 5.3):
• tunica adventitia or outer layer of fibrous tissue
• tunica media or middle layer of smooth muscle and elastic tissue
• tunica intima or inner lining of squamous epithelium called endothelium.
The amount of muscular and elastic tissue varies in the arteries depending upon their size and function. In the large arteries, including the aorta, sometimes called elastic arteries, the tunica media contains more elastic tissue and less smooth muscle. This allows the vessel wall to stretch, absorbing the pressure wave generated by the heart as it beats. These proportions gradually change as the arteries branch many times and become smaller until in the arterioles (the smallest arteries) the tunica media consists almost entirely of smooth muscle. This enables their diameter to be precisely controlled, which regulates the pressure within them. Systemic blood pressure is mainly determined by the resistance these tiny arteries offer to blood flow, and for this reason they are called resistance vessels.
Arteries have thicker walls than veins to withstand the high pressure of arterial blood.
Anastomoses and end-arteries
Anastomoses are arteries that form a link between main arteries supplying an area, e.g. the arterial supply to the palms of the hand (p. 107) and soles of the feet, the brain, the joints and, to a limited extent, the heart muscle. If one artery supplying the area is occluded, anastomotic arteries provide a collateral circulation. This is most likely to provide an adequate blood supply when the occlusion occurs gradually, giving the anastomotic arteries time to dilate.
An end-artery is an artery that is the sole source of blood to a tissue, e.g. the branches from the circulus arteriosus (circle of Willis) in the brain or the central artery to the retina of the eye. When an end-artery is occluded the tissues it supplies die because there is no alternative blood supply.
Capillaries and sinusoids
The smallest arterioles break up into a number of minute vessels called capillaries. Capillary walls consist of a single layer of endothelial cells sitting on a very thin basement membrane, through which water and other small molecules can pass. Blood cells and large molecules such as plasma proteins do not normally pass through capillary walls. The capillaries form a vast network of tiny vessels that link the smallest arterioles to the smallest venules. Their diameter is approximately that of an erythrocyte (7 µm). The capillary bed is the site of exchange of substances between the blood and the tissue fluid, which bathes the body cells and, with the exception of those on the skin surface and in the cornea of the eye, every body cell lies close to a capillary.
Entry to capillary beds is guarded by rings of smooth muscle (precapillary sphincters) that direct blood flow. Hypoxia (low levels of oxygen in the tissues), or high levels of tissue wastes, indicating high levels of activity, dilate the sphincters and increase blood flow through the affected beds.
In certain places, including the liver (p. 309) and bone marrow, the capillaries are significantly wider and leakier than normal. These capillaries are called sinusoids and because their walls are incomplete and their lumen is much larger than usual, blood flows through them more slowly under less pressure and can come directly into contact with the cells outside the sinusoid wall. This allows much faster exchange of substances between the blood and the tissues, useful, for example, in the liver, which regulates the composition of blood arriving from the gastrointestinal tract.
Capillary refill time
When an area of skin is pressed firmly with a finger, it turns white (blanches) because the blood in the capillaries under the finger has been squeezed out. Normally it should take less than two seconds for the capillaries to refill once the finger is removed, and for the skin to turn pink again. Although the test may produce unreliable results, particularly in adults, its use in children can be useful and a prolonged capillary refill time suggests poor perfusion or dehydration.
Veins and venules
Veins return blood at low pressure to the heart. The walls of the veins are thinner than arteries but have the same three layers of tissue (Fig. 5.3). They are thinner because there is less muscle and elastic tissue in the tunica media, as veins carry blood at a lower pressure than arteries. When cut, the veins collapse while the thicker-walled arteries remain open. When an artery is cut blood spurts at high pressure while a slower, steady flow of blood escapes from a vein.
Some veins possess valves, which prevent backflow of blood, ensuring that it flows towards the heart (Fig. 5.4). They are formed by a fold of tunica intima and strengthened by connective tissue. The cusps are semilunar in shape with the concavity towards the heart. Valves are abundant in the veins of the limbs, especially the lower limbs where blood must travel a considerable distance against gravity when the individual is standing. They are absent in very small and very large veins in the thorax and abdomen. Valves are assisted in maintaining one-way flow by skeletal muscles surrounding the veins (p. 95).
Figure 5.4 Interior of a vein. A. The valves and cusps. B. The direction of blood flow through a valve.
The smallest veins are called venules.
Veins are called capacitance vessels because they are distensible, and therefore have the capacity to hold a large proportion of the body’s blood. At any one time, about two-thirds of the body’s blood is in the venous system. This allows the vascular system to absorb (to an extent) sudden changes in blood volume, such as in haemorrhage; the veins can constrict, helping to prevent a sudden fall in blood pressure.
Blood supply
The outer layers of tissue of thick-walled blood vessels receive their blood supply via a network of blood vessels called the vasa vasorum. Thin-walled vessels and the endothelium of the others receive oxygen and nutrients by diffusion from the blood passing through them.
Control of blood vessel diameter
The smooth muscle in the tunica media of veins and arteries is supplied by nerves of the autonomic nervous system. These nerves arise from the vasomotor centre in the medulla oblongata and they change the diameter of blood vessels, controlling the volume of blood they contain.
The blood vessels most closely regulated by this nervous mechanism are the arterioles, since they contain proportionately more smooth muscle in their walls than any other blood vessel. The walls of large arteries such as the aorta contain mainly elastic tissue and so they tend to passively expand and recoil, depending on how much blood is passing through them. Veins also respond to nerve stimulation, although they have only a little smooth muscle in their tunica media.
Blood vessel diameter and blood flow
Resistance to flow of fluids along a tube is determined by three factors: the diameter of the tube; the length of the tube; and the viscosity of the fluid. The most important factor determining how easily the blood flows through blood vessels is the first of these variables, that is, the diameter of the resistance vessels (the peripheral resistance). The length of the vessels and viscosity of blood also contribute to peripheral resistance, but in health these are constant and are therefore not significant determinants of changes in blood flow. Peripheral resistance is a major factor in blood pressure regulation, which is further discussed on page 96.
Blood vessel diameter is regulated by the smooth muscle of the tunica media, which is supplied by sympathetic nerves of the autonomic nervous system (p. 173). There is no parasympathetic nerve supply to most blood vessels and therefore the diameter of the vessel lumen and the tone of the smooth muscle are determined by the degree of sympathetic activity. Sympathetic activity generally constricts blood vessel smooth muscle and therefore narrows the vessel (vasoconstriction), increasing the pressure inside. A degree of resting sympathetic activity maintains a constant baseline tone in the vessel wall and prevents pressure falling too low (Fig. 5.5). Decreased nerve stimulation relaxes the smooth muscle, thinning the vessel wall and enlarging the lumen (vasodilation). This results in increased blood flow under less pressure.
Constant adjustment of blood vessel diameter helps to regulate peripheral resistance and systemic blood pressure.
Although most arterioles respond to sympathetic stimulation with vasoconstriction, the response is much less marked in some arteriolar beds, e.g. in skeletal muscle and the brain. This is important so that in a stress response, such as the flight or fight response (p. 176), when sympathetic activity is very high, these essential tissues receive the extra oxygen and nutrients they need.
Local regulation of blood flow
Tissues’ oxygen and nutrient requirements vary depending on their activities, so it is important that blood flow is regulated locally to ensure that blood flow matches tissue needs. The ability of an organ to control its own blood flow according to need is called autoregulation. Some organs, including the central nervous system, liver and kidneys receive proportionately higher blood flow as a matter of course. Other tissues, such as resting skeletal muscle, receive much less, but their blood supply can increase by as much as 20-fold during heavy exercise. Other examples include blood flow through the gastrointestinal tract increasing after a meal to allow for increased activity in the tract, and adjustments to blood flow through the skin in the control of body temperature (p. 367). Blood flow through individual organs is increased by vasodilation of the vessels supplying it, and decreased through vasoconstriction. The main mechanisms associated with this local control of blood flow include:
• release of vasodilator chemicals. Inflamed and metabolically active tissues produce a number of vasodilators, which increase blood supply to the area. One important vasodilator is nitric oxide, which is very short lived, but which is important in opening up the larger arteries supplying an organ. Other agents include substances released in the inflammatory response, such as histamine and bradykinin (p. 378)
• action of vasoconstrictors. The sympathetic hormone adrenaline (epinephrine), released from the adrenal medulla, is a powerful vasoconstrictor. Others include angiotensin 2 (p. 343).
Capillary exchange
Exchange of gases
Internal respiration (Fig. 5.6) is the process by which gases are exchanged between capillary blood and local body cells.
Oxygen is carried from the lungs to the tissues in combination with haemoglobin (p. 66) as oxyhaemoglobin. Exchange in the tissues takes place between blood at the arterial end of the capillaries and the tissue fluid and then between the tissue fluid and the cells. Oxygen diffuses down its concentration gradient, from the oxygen-rich arterial blood, into the tissues, where oxygen levels are lower because of constant tissue consumption.
Oxyhaemoglobin is an unstable compound and breaks up (dissociates) easily to liberate oxygen. Factors that increase dissociation are discussed on page 66.
Carbon dioxide is one of the waste products of cell metabolism and, towards the venous end of the capillary, it diffuses into the blood down the concentration gradient. Blood transports carbon dioxide to the lungs for excretion by three different mechanisms:
• dissolved in the water of the blood plasma – 7%
• in chemical combination with sodium in the form of sodium bicarbonate – 70%
Exchange of other substances
The nutrients, including glucose, amino acids, fatty acids, vitamins and mineral salts required by all body cells are transported round the body in the blood plasma. They diffuse through the semipermeable capillary walls into the tissues (Fig. 5.7). Water exchanges freely between the plasma and tissue fluid by osmosis. Diffusion and osmosis are described on p. 29.
Capillary fluid dynamics
The two main forces determining overall fluid movement across the capillary wall are the hydrostatic pressure (blood pressure), which tends to push fluid out of the bloodstream, and the osmotic pressure of the blood, which tends to pull it back in, and is due mainly to the presence of plasma proteins, especially albumin (Fig. 5.8).
At the arterial end, the hydrostatic pressure is about 5 kPa (35 mmHg), and the opposing osmotic pressure of the blood is only 3 kPa (25 mmHg). The overall force at the arterial end of the capillary therefore drives fluid out of the capillary and into the tissue spaces. This net loss of fluid from the bloodstream must be reclaimed in some way.
At the venous end of the capillary, the situation is reversed. Blood flow is slower than at the arterial end because the hydrostatic pressure drops along the capillary to only 2 kPa (15 mmHg). The osmotic pressure remains unchanged at 3 kPa (25 mmHg) and, because this now exceeds hydrostatic pressure, fluid moves back into the capillary.
This transfer of substances, including water, to the tissue spaces is a dynamic process. As blood flows slowly through the large network of capillaries from the arterial to the venous end, there is constant change. Not all the water and cell waste products return to the blood capillaries. Of the 24 litres or so of fluid that moves out of the blood across capillary walls every day, only about 21 litres returns to the bloodstream at the venous end of the capillary bed. The excess is drained away from the tissue spaces in the minute lymph capillaries which originate as blind-end tubes with walls similar to, but more permeable than, those of the blood capillaries (Fig. 5.7). Extra tissue fluid and some cell waste materials enter the lymph capillaries and are eventually returned to the bloodstream (Ch. 6).
Heart
The heart is a roughly cone-shaped hollow muscular organ. It is about 10 cm long and is about the size of the owner’s fist. It weighs about 225 g in women and is heavier in men (about 310 g).
Position
5.2
The heart lies in the thoracic cavity (Fig. 5.9) in the mediastinum (the space between the lungs). It lies obliquely, a little more to the left than the right, and presents a base above, and an apex below. The apex is about 9 cm to the left of the midline at the level of the 5th intercostal space, i.e. a little below the nipple and slightly nearer the midline. The base extends to the level of the 2nd rib.
Structure
The heart wall
The heart wall is composed of three layers of tissue (Fig. 5.11A): pericardium, myocardium and endocardium.
Figure 5.11 Tissues of the heart wall. A. Layers of the heart wall: endocardium, myocardium and pericardium. B. Cardiac muscle tissue. C. Coloured electron micrograph of cardiac muscle tissue.
Pericardium
The pericardium is the outermost layer and is made up of two sacs. The outer sac (the fibrous pericardium) consists of fibrous tissue and the inner (the serous pericardium) of a continuous double layer of serous membrane.
The fibrous pericardium is continuous with the tunica adventitia of the great blood vessels above and is adherent to the diaphragm below. Its inelastic, fibrous nature prevents overdistension of the heart.
The outer layer of the serous pericardium, the parietal pericardium, lines the fibrous pericardium. The inner layer, the visceral pericardium, which is continuous with the parietal pericardium, is adherent to the heart muscle. A similar arrangement of a double membrane forming a closed space is seen also with the pleura, the membrane enclosing the lungs (see Fig. 10.15, p. 250).
The serous membrane consists of flattened epithelial cells. It secretes serous fluid, called pericardial fluid, into the space between the visceral and parietal layers, which allows smooth movement between them when the heart beats. The space between the parietal and visceral pericardium is only a potential space. In health the two layers lie closely together, with only the thin film of pericardial fluid between them.
Myocardium
The myocardium is composed of specialised cardiac muscle found only in the heart (Fig. 5.11B and C). It is striated, like skeletal muscle, but is not under voluntary control. Each fibre (cell) has a nucleus and one or more branches. The ends of the cells and their branches are in very close contact with the ends and branches of adjacent cells. Microscopically these ‘joints’, or intercalated discs, are thicker, darker lines than the striations. This arrangement gives cardiac muscle the appearance of being a sheet of muscle rather than a very large number of individual cells. Because of the end-to-end continuity of the fibres, each one does not need to have a separate nerve supply. When an impulse is initiated it spreads from cell to cell via the branches and intercalated discs over the whole ‘sheet’ of muscle, causing contraction. The ‘sheet’ arrangement of the myocardium enables the atria and ventricles to contract in a coordinated and efficient manner.
Running through the myocardium is also the network of specialised conducting fibres responsible for transmitting the heart’s electrical signals. The myocardium is thickest at the apex and thins out towards the base (Fig. 5.12). This reflects the amount of work each chamber contributes to the pumping of blood. It is thickest in the left ventricle, which has the greatest workload.
Specialised muscle cells in the walls of the atria secrete atrial natriuretic peptide (ANP, p. 228).
Fibrous tissue in the heart.
The myocardium is supported by a network of fine fibres that run through all the heart muscle. This is called the fibrous skeleton of the heart. In addition, the atria and the ventricles are separated by a ring of fibrous tissue, which does not conduct electrical impulses. Consequently, when a wave of electrical activity passes over the atrial muscle, it can only spread to the ventricles through the conducting system that bridges the fibrous ring from atria to ventricles (p. 90).
Endocardium
This lines the chambers and valves of the heart. It is a thin, smooth membrane to ensure smooth flow of blood through the heart. It consists of flattened epithelial cells, and it is continuous with the endothelium lining the blood vessels.
Interior of the heart
5.3, 5.4
The heart is divided into a right and left side by the septum (Fig. 5.12), a partition consisting of myocardium covered by endocardium. After birth, blood cannot cross the septum from one side to the other. Each side is divided by an atrioventricular valve into the upper atrium and the ventricle below. The atrioventricular valves are formed by double folds of endocardium strengthened by a little fibrous tissue. The right atrioventricular valve (tricuspid valve) has three flaps or cusps and the left atrioventricular valve (mitral valve, Fig. 5.13) has two cusps. Flow of blood in the heart is one way; blood enters the heart via the atria and passes into the ventricles below.
Figure 5.13 The left atrioventricular (mitral) valve. A. Valve open. B. Valve closed. C. Photograph of the chordae tendinae.
The valves between the atria and ventricles open and close passively according to changes in pressure in the chambers (Fig. 5.13A and B). They open when the pressure in the atria is greater than that in the ventricles. During ventricular systole (contraction) the pressure in the ventricles rises above that in the atria and the valves snap shut, preventing backward flow of blood. The valves are prevented from opening upwards into the atria by tendinous cords, called chordae tendineae (Fig. 5.13C), which extend from the inferior surface of the cusps to little projections of myocardium into the ventricles, covered with endothelium, called papillary muscles (Fig. 5.13).
Flow of blood through the heart (Fig. 5.14)
5.5
The two largest veins of the body, the superior and inferior venae cavae, empty their contents into the right atrium. This blood passes via the right atrioventricular valve into the right ventricle, and from there is pumped into the pulmonary artery or trunk (the only artery in the body which carries deoxygenated blood). The opening of the pulmonary artery is guarded by the pulmonary valve, formed by three semilunar cusps. This valve prevents the backflow of blood into the right ventricle when the ventricular muscle relaxes. After leaving the heart the pulmonary artery divides into left and right pulmonary arteries, which carry the venous blood to the lungs where exchange of gases takes place: carbon dioxide is excreted and oxygen is absorbed.
Two pulmonary veins from each lung carry oxygenated blood back to the left atrium. Blood then passes through the left atrioventricular valve into the left ventricle, and from there it is pumped into the aorta, the first artery of the general circulation. The opening of the aorta is guarded by the aortic valve, formed by three semilunar cusps (Fig. 5.15).
From this sequence of events it can be seen that the blood passes from the right to the left side of the heart via the lungs, or pulmonary circulation (Fig. 5.16). However, it should be noted that both atria contract at the same time and this is followed by the simultaneous contraction of both ventricles.
The muscle layer of the walls of the atria is thinner than that of the ventricles (Fig. 5.12). This is consistent with the amount of work they do. The atria, usually assisted by gravity, pump the blood only through the atrioventricular valves into the ventricles, whereas the more powerful ventricles pump the blood to the lungs and round the whole body.
The pulmonary trunk leaves the heart from the upper part of the right ventricle, and the aorta leaves from the upper part of the left ventricle.
Blood supply to the heart (the coronary circulation)
5.6
Arterial supply (Fig. 5.17).
The heart is supplied with arterial blood by the right and left coronary arteries, which branch from the aorta immediately distal to the aortic valve (Figs 5.15 and 5.17). The coronary arteries receive about 5% of the blood pumped from the heart, although the heart comprises a small proportion of body weight. This large blood supply, of which a large proportion goes to the left ventricle, highlights the importance of the heart to body function. The coronary arteries traverse the heart, eventually forming a vast network of capillaries.
Venous drainage.
Most of the venous blood is collected into a number of cardiac veins that join to form the coronary sinus, which opens into the right atrium. The remainder passes directly into the heart chambers through little venous channels.
Conducting system of the heart (Fig. 5.18)
5.7
The heart possesses the property of autorhythmicity, which means it generates its own electrical impulses and beats independently of nervous or hormonal control, i.e. it is not reliant on external mechanisms to initiate each heartbeat. However, it is supplied with both sympathetic and parasympathetic nerve fibres, which increase and decrease respectively the intrinsic heart rate. In addition, the heart responds to a number of circulating hormones, including adrenaline (epinephrine) and thyroxine.
Small groups of specialised neuromuscular cells in the myocardium initiate and conduct impulses, causing coordinated and synchronised contraction of the heart muscle.
Sinoatrial node (SA node)
This small mass of specialised cells lies in the wall of the right atrium near the opening of the superior vena cava.
The sinoatrial cells generate these regular impulses because they are electrically unstable. This instability leads them to discharge (depolarise) regularly, usually between 60 and 80 times a minute. This depolarisation is followed by recovery (repolarisation), but almost immediately their instability leads them to discharge again, setting the heart rate. Because the SA node discharges faster than any other part of the heart, it normally sets the heart rate and is called the pacemaker of the heart. Firing of the SA node triggers atrial contraction.
Atrioventricular node (AV node)
This small mass of neuromuscular tissue is situated in the wall of the atrial septum near the atrioventricular valves. Normally, the AV node merely transmits the electrical signals from the atria into the ventricles. There is a delay here; the electrical signal takes 0.1 of a second to pass through into the ventricles. This allows the atria to finish contracting before the ventricles start.
The AV node also has a secondary pacemaker function and takes over this role if there is a problem with the SA node itself, or with the transmission of impulses from the atria. Its intrinsic firing rate, however, is slower than that set by the SA node (40–60 beats per minute).
Atrioventricular bundle (AV bundle or bundle of His)
This mass of specialised fibres originates from the AV node. The AV bundle crosses the fibrous ring that separates atria and ventricles then, at the upper end of the ventricular septum, it divides into right and left bundle branches. Within the ventricular myocardium the branches break up into fine fibres, called the Purkinje fibres. The AV bundle, bundle branches and Purkinje fibres transmit electrical impulses from the AV node to the apex of the myocardium where the wave of ventricular contraction begins, then sweeps upwards and outwards, pumping blood into the pulmonary artery and the aorta.
Nerve supply to the heart
As mentioned earlier, the heart is influenced by autonomic (sympathetic and parasympathetic) nerves originating in the cardiovascular centre in the medulla oblongata.
The vagus nerve (parasympathetic) supplies mainly the SA and AV nodes and atrial muscle. Vagal stimulation reduces the rate at which impulses are produced, decreasing the rate and force of the heartbeat.
Sympathetic nerves supply the SA and AV nodes and the myocardium of atria and ventricles, and stimulation increases the rate and force of the heartbeat.
The cardiac cycle
At rest, the healthy adult heart is likely to beat at a rate of 60–80 beats per minute (b.p.m.). During each heartbeat, or cardiac cycle (Fig. 5.19), the heart contracts (systole) and then relaxes (diastole).
Stages of the cardiac cycle
Taking 74 b.p.m. as an example, each cycle lasts about 0.8 of a second and consists of:
• atrial systole – contraction of the atria
• ventricular systole – contraction of the ventricles
• complete cardiac diastole – relaxation of the atria and ventricles.
It does not matter at which stage of the cardiac cycle a description starts. For convenience the period when the atria are filling has been chosen.
The superior vena cava and the inferior vena cava transport deoxygenated blood into the right atrium at the same time as the four pulmonary veins bring oxygenated blood into the left atrium. The atrioventricular valves are open and blood flows passively through to the ventricles. The SA node triggers a wave of contraction that spreads over the myocardium of both atria, emptying the atria and completing ventricular filling (atrial systole 0.1 s;Fig. 5.19A). When the electrical impulse reaches the AV node it is slowed down, delaying atrioventricular transmission. This delay means that the mechanical result of atrial stimulation, atrial contraction, lags behind the electrical activity by a fraction of a second. This allows the atria to finish emptying into the ventricles before the ventricles begin to contract. After this brief delay, the AV node triggers its own electrical impulse, which quickly spreads to the ventricular muscle via the AV bundle, the bundle branches and Purkinje fibres. This results in a wave of contraction which sweeps upwards from the apex of the heart and across the walls of both ventricles pumping the blood into the pulmonary artery and the aorta (ventricular systole 0.3 s; Fig. 5.19B). The high pressure generated during ventricular contraction forces the atrioventricular valves to close, preventing backflow of blood into the atria.
After contraction of the ventricles there is complete cardiac diastole, a period of 0.4 seconds, when atria and ventricles are relaxed. During this time the myocardium recovers ready for the next heartbeat, and the atria refill ready for the next cycle (Fig. 5.19C).
The valves of the heart and of the great vessels open and close according to the pressure within the chambers of the heart. The AV valves are open while the ventricular muscle is relaxed during atrial filling and systole. When the ventricles contract there is a rapid increase in the pressure in these chambers, and when it rises above atrial pressure the atrioventricular valves close. When the ventricular pressure rises above that in the pulmonary artery and in the aorta, the pulmonary and aortic valves open and blood flows into these vessels. When the ventricles relax and the pressure within them falls, the reverse process occurs. First the pulmonary and aortic valves close, then the atrioventricular valves open and the cycle begins again. This sequence of opening and closing valves ensures that the blood flows in only one direction.
Heart sounds
The individual is not usually conscious of their heartbeat, but if the ear, or the diaphragm of a stethoscope, is placed on the chest wall a little below the left nipple and slightly nearer the midline the heartbeat can be heard (Fig. 5.9).
There are four heart sounds, each corresponding to a particular event in the cardiac cycle. The first two are most easily distinguished, and sound through the stethoscope like ‘lub dup’. The first sound, ‘lub’, is fairly loud and is due to the closure of the atrioventricular valves. This corresponds with the start of ventricular systole. The second sound, ‘dup’, is softer and is due to the closure of the aortic and pulmonary valves. This corresponds with ventricular diastole.
Electrical changes in the heart
5.8
The body tissues and fluids conduct electricity well, so the electrical activity in the heart can be recorded on the skin surface using electrodes positioned on the limbs and/or the chest. This recording, called an electrocardiogram (ECG) shows the spread of the electrical signal generated by the SA node as it travels through the atria, the AV node and the ventricles. The normal ECG tracing shows five waves which, by convention, have been named P, Q, R, S and T (Fig. 5.20).
Figure 5.20 Electrocardiogram of one cardiac cycle. A, B and C correspond to the phases of the cardiac cycle shown in Figure 5.19.
The P wave arises when the impulse from the SA node sweeps over the atria (atrial depolarisation).
The QRS complex represents the very rapid spread of the impulse from the AV node through the AV bundle and the Purkinje fibres and the electrical activity of the ventricular muscle (ventricular depolarisation). Note the delay between the completion of the P wave and the onset of the QRS complex. This represents the conduction of the impulse through the AV node (p. 92), which is much slower than conduction elsewhere in the heart, and allows atrial contraction to finish completely before ventricular contraction starts.
The T wave represents the relaxation of the ventricular muscle (ventricular repolarisation). Atrial repolarisation occurs during ventricular contraction, and so is not seen because of the larger QRS complex.
The ECG described above originates from the SA node and is called sinus rhythm. The rate of sinus rhythm is 60–100 b.p.m. A faster heart rate is called tachycardia and a slower heart rate, bradycardia.
By examining the pattern of waves and the time interval between cycles and parts of cycles, information about the state of the myocardium and the cardiac conduction system is obtained.
Cardiac output
The cardiac output is the amount of blood ejected from each ventricle every minute. The amount expelled by each contraction of each ventricle is the stroke volume. Cardiac output is expressed in litres per minute (L/min) and is calculated by multiplying the stroke volume by the heart rate (measured in beats per minute):
In a healthy adult at rest, the stroke volume is approximately 70 mL and if the heart rate is 72 per minute, the cardiac output is 5 L/minute. This can be greatly increased to meet the demands of exercise to around 25 L/minute, and in athletes up to 35 L/minute. This increase during exercise is called the cardiac reserve.
When increased blood supply is needed to meet increased tissue requirements of oxygen and nutrients, heart rate and/or stroke volume can be increased (see Box 5.2).
Stroke volume
The stroke volume is determined by the volume of blood in the ventricles immediately before they contract, i.e. the ventricular end-diastolic volume (VEDV), sometimes called preload. In turn, preload depends on the amount of blood returning to the heart through the superior and inferior venae cavae (the venous return). Increased preload leads to stronger myocardial contraction, and more blood is expelled. In turn the stroke volume and cardiac output rise. In this way, the heart, within physiological limits, always pumps out all the blood that it receives, allowing it to adjust cardiac output to match body needs. This capacity to increase the stroke volume with increasing preload is finite, and when the limit is reached, i.e. venous return to the heart exceeds cardiac output (i.e. more blood is arriving in the atria than the ventricles can pump out), cardiac output decreases and the heart begins to fail (p. 126). Other factors that increase the force and rate of myocardial contraction include increased sympathetic nerve activity and circulating hormones, e.g. adrenaline (epinephrine), noradrenaline (norepinephrine) and thyroxine.
Arterial blood pressure.
This affects the stroke volume as it creates resistance to blood being pumped from the ventricles into the great arteries. This resistance (sometimes called afterload) is determined by the distensibility, or elasticity, of the large arteries and the peripheral resistance of arterioles (p. 85). Increasing afterload increases the workload of the ventricles, because it increases the pressure against which they have to pump. This may actually reduce stroke volume if systemic blood pressure becomes significantly higher than normal.
Blood volume.
This is normally kept constant by the kidneys. Should blood volume fall, e.g. through sudden haemorrhage, this can cause stroke volume, cardiac output and venous return to fall. However, the body’s compensatory mechanisms (p. 96) will tend to return these values towards normal, unless the blood loss is too sudden or severe for compensation (see Shock, p. 118).
Venous return
Venous return is the major determinant of cardiac output and, normally, the heart pumps out all blood returned to it. The force of contraction of the left ventricle ejecting blood into the aorta is not sufficient to push the blood through the arterial and venous circulation and back to the heart. Other factors are involved.
The position of the body.
Gravity assists venous return from the head and neck when standing or sitting and offers less resistance to venous return from the lower parts of the body when lying flat.
Muscular contraction.
Backflow of blood in veins of the limbs, especially when standing, is prevented by valves (Fig. 5.4). The contraction of skeletal muscles surrounding the deep veins compresses them, pushing blood towards the heart (Fig. 5.21). In the lower limbs, this is called the skeletal muscle pump.
The respiratory pump.
During inspiration, the expansion of the chest creates a negative pressure within the thorax, assisting flow of blood towards the heart. In addition, when the diaphragm descends during inspiration, the increased intra-abdominal pressure pushes blood towards the heart.
Heart rate
The heart rate is a major determinant of cardiac output. If heart rate rises, cardiac output increases, and if it falls, cardiac output falls too. The main factors determining heart rate are outlined below.
Autonomic nervous system.
The intrinsic rate at which the heart beats is a balance between sympathetic and parasympathetic activity and this is the most important factor in determining heart rate.
Circulating chemicals.
The hormones adrenaline (epinephrine) and noradrenaline (norepinephrine), secreted by the adrenal medulla, have the same effect as sympathetic stimulation, i.e. they increase the heart rate. Other hormones, including thyroxine, increase heart rate. Hypoxia and elevated carbon dioxide levels stimulate heart rate. Electrolyte imbalances may affect it, e.g. hyperkalaemia depresses cardiac function and leads to bradycardia (slow heart rate). Some drugs, such as β-receptor antagonists (e.g. atenolol) used in hypertension, can also cause bradycardia.
Position.
When upright, the heart rate is usually faster than when lying down.
Exercise.
Active muscles need more blood than resting muscles and this is achieved by an increased heart rate and local vasodilation.
Emotional states.
During excitement, fear or anxiety the heart rate is increased. Other effects mediated by the sympathetic nervous system may be present (see Fig. 7.43, p. 174).
Gender.
The heart rate is faster in women than men.
Age.
In babies and small children the heart rate is more rapid than in older children and adults.
Temperature.
The heart rate rises and falls with body temperature.
Blood pressure
Blood pressure is the force or pressure that the blood exerts on the walls of blood vessels. Systemic arterial blood pressure maintains the essential flow of blood into and out of the organs of the body. Keeping blood pressure within normal limits is very important. If it becomes too high, blood vessels can be damaged, causing clots or bleeding from sites of blood vessel rupture. If it falls too low, then blood flow through tissue beds may be inadequate. This is particularly dangerous for essential organs such as the heart, brain or kidneys.
The systemic arterial blood pressure, usually called simply arterial blood pressure, is the result of the discharge of blood from the left ventricle into the already full aorta.
Blood pressure varies according to the time of day, the posture, gender and age of the individual. Blood pressure falls at rest and during sleep. It increases with age and is usually higher in women than in men.
Systolic and diastolic pressures.
When the left ventricle contracts and pushes blood into the aorta, the pressure produced within the arterial system is called the systolic blood pressure. In adults it is about 120 mmHg or 16 kPa.
In complete cardiac diastole when the heart is resting following the ejection of blood, the pressure within the arteries is much lower and is called diastolic blood pressure. In an adult this is about 80 mmHg or 11 kPa. The difference between systolic and diastolic blood pressures is the pulse pressure.
Arterial blood pressure (BP) is measured with a sphygmomanometer and is usually expressed with the systolic pressure written above the diastolic pressure:
Elasticity of arterial walls.
There is a considerable amount of elastic tissue in the arterial walls, especially in large arteries. Therefore, when the left ventricle ejects blood into the already full aorta, the aorta expands to accommodate it, and then recoils because of the elastic tissue in the wall. This pushes the blood forwards, into the systemic circulation. This distension and recoil occurs throughout the arterial system. During cardiac diastole the elastic recoil of the arteries maintains the diastolic pressure.
Factors determining blood pressure
Blood pressure is determined by cardiac output and peripheral resistance. Change in either of these parameters tends to alter systemic blood pressure, although the body’s compensatory mechanisms usually adjust for any significant change.
Cardiac output
Cardiac output is determined by the stroke volume and the heart rate (p. 94
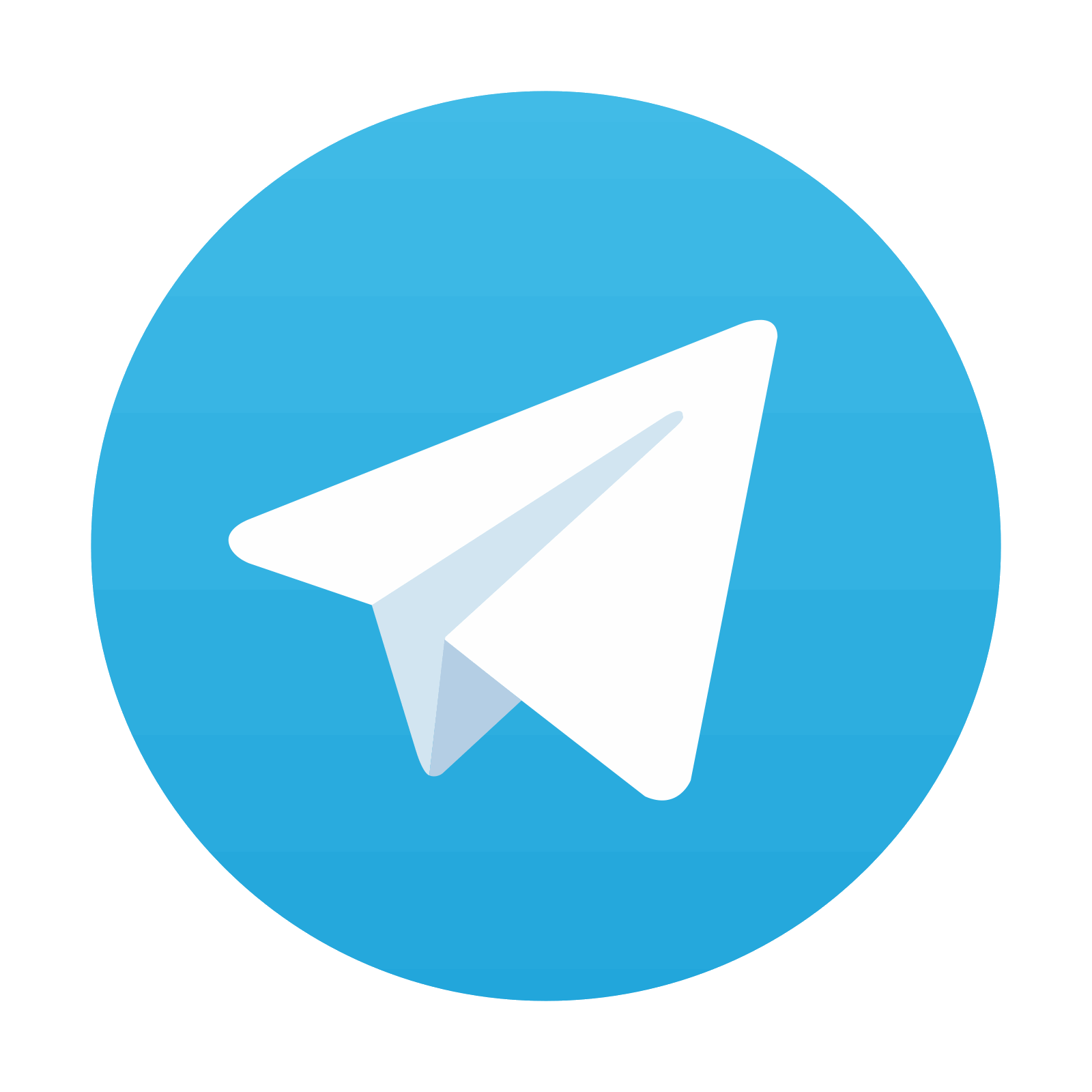
Stay updated, free articles. Join our Telegram channel
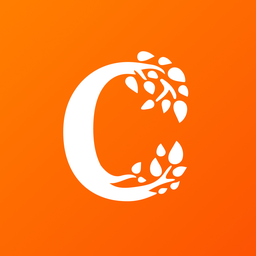
Full access? Get Clinical Tree
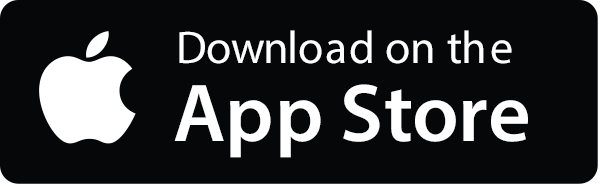
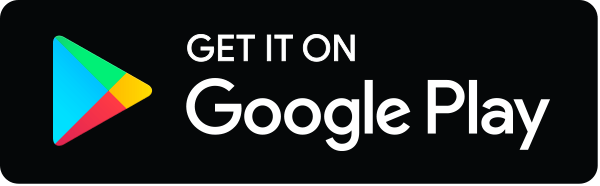
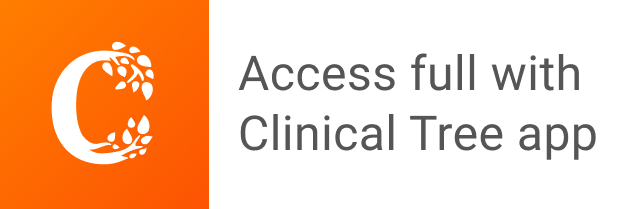