“Do you mean to say you think you’ve discovered an infectious disease of bacteria, and you haven’t told me about it? My dear boy, I don’t believe you quite realize that you may have hit on the supreme way to kill pathogenic bacteria … And you didn’t tell me!”
—Sinclair Lewis, Arrowsmith (1924)
20.1 History of Bacteriophage Research
Bacteriophages are viruses that infect bacteria. The term bacteriophage is a combination of the word bacteria and the Greek word phagein, meaning “to eat.” The term bacteriophage is often abbreviated as phage. Phages were discovered after the recognition of bacterial hosts in the 1880s, a period known as the golden age of microbiology. British bacteriologist Frederick W. Twort authored a peer-reviewed journal article published in 1915 that is considered to be the birth of modern bacteriophage research. He reported an observation called glassy transformation. At the time, Twort was trying to cultivate vaccinia viruses on agar medium in the absence of host cells. He inoculated the solid medium with unfiltered pustule fluid used in smallpox vaccinations. Rather than vaccinia virus, though, micrococci colonies grew on the solid medium, and they appeared watery or glassy in some areas. Twort collected the watery colonies, which could not be grown on new medium, and filtered them with a Chamberland ultrafilter. The filtrate was added to fresh cultures of micrococci, causing them to undergo glassy transformation. He concluded that a filterable infectious agent killed the bacteria, and that in the process the agent multiplied itself, thus causing the glassy appearance. Twort did not state that it was a bacterial virus despite the fact that it was known that filterable agents infected plants and animals. The scientific community did not acknowledge Twort’s published report on glassy transformation for 5 years.
French-Canadian Felix d’Herelle is credited as the sole discoverer of bacteriophages (FIGURE 20-1). In a 1917 paper, he described the lysis of dysentery-causing bacteria grown in liquid medium. He hypothesized that “an invisible antagonist”—a bacteriophage—could pass through a Chamberland ultrafilter and was responsible for lysis of the dysentery bacteria. d’Herelle did not cite Twort’s research in his research report. He continued research in two directions: (1) determining the biological nature of bacteriophages and (2) exploring the use of bacteriophages as a therapy to treat bacterial infections in a pre-antibiotic era.

FIGURE 20-1 Felix d’Herelle (1873–1949) is credited with the discovery of bacteriophages and is considered the father of bacteriophage therapy.
d’Herelle believed that bacteriophages were an agent of immunity in fighting infectious diseases, a conclusion he reached by observing that the number of bacteriophages increased in patients recovering from bacterial dysentery and typhoid. He speculated that the lysis of bacteria by specific bacteriophages formed the mechanism of recovery. This idea supported his efforts to use bacteriophage therapy to treat a wide variety of bacterial diseases. The majority of the scientific world failed to accept the fact that bacteriophages were filterable viruses of bacteria. Instead, the leading theory regarding bacterial lysis was based on Jules Bordet’s view that endogenous autolytic enzymes caused bacteria to be lysed during infection. Not until the early 1940s did d’Herelle’s bacteriophage theory become universally accepted.
d’Herelle was quick to realize the therapeutic potential of bacteriophages. During the summer of 1919 in France, he attempted to use bacteriophages in field trials to control an epidemic of chicken typhoid caused by the bacterium Salmonella gallinarum. He inoculated chickens either orally or by injection with bacteriophages, and the overall results suggested that bacteriophage-treated flocks suffered fewer deaths and shorter epidemics that did not reoccur. He also conducted field trials in Indochina during an outbreak of highly fatal water buffalo pasteurellosis (sometimes referred to in French as bar-bone disease). Once again inoculations appeared successful. The positive results motivated d’Herelle to conduct human trials in the 1920s, a time when human trials were scientifically and ethically crude and not very safe by today’s standards.
To prove that bacteriophage preparations (Shiga-bacteriophage lysates) were safe for human experiments, d’Herelle injected himself, his family members, and his coworkers. No harmful effects were observed. He applied this treatment to a 12-year-old boy suffering from a confirmed case of bacterial dysentery. The boy’s symptoms disappeared after one dose, and he fully recovered after a few days. Based on this success, three more dysentery patients received similar treatment. All of them were cured.
d’Herelle’s most notorious bacteriophage therapy experiments took place at the League of Nations quarantine station in Alexandria, Egypt. Four patients on a ship passing through the Suez Canal were suffering from laboratory-confirmed bubonic plague. d’Herelle injected all four individuals with antiplague bacteriophages into the bubos present in their lymph nodes, and all four patients recovered rapidly. This extraordinary result was reported in 1925 in the French medical journal Presse Medicale. After the report, the British government invited d’Herelle to work at the Haffkine Institute in Bombay on bacteriophage therapies for plague and cholera in India. Cholera epidemics posed a major concern because they occurred regularly during religious festivals and pilgrimages in India. Reports from India during the 1920s and 1930s were positive regarding the oral administration of cholera-specific bacteriophages in patients. Bacteriophage therapy reduced the overall severity of symptoms and mortality from the disease.
d’Herelle traveled the world continuing bacteriophage therapy as a means to control cholera outbreaks. He accepted an appointment as a professor of protobiology at Yale University in 1928 and went on to play a vital role in the establishment of a bacteriophage institute in Tbilisi, Georgia (then a Soviet republic, today the Republic of Georgia), in 1934. The institute exists today as the George Eliava Institute of Bacteriophage, Microbiology, and Virology. Bacteriophage therapy was popularized by Sinclair Lewis’s Pulitzer Prize–winning 1924 novel Arrowsmith. The main character, a young physician named Martin Arrowsmith, travels to the West Indies to use bacteriophages as a therapy against bubonic plague.
For several reasons, nearly all bacteriologists abandoned bacteriophage therapy in the 1930s. For one, published reports on bacteriophage therapy contained both negative and positive conclusions. It appeared to be a “hit or miss” therapy, and the biology of bacteriophages was poorly understood. The main reason, though, for the abandonment of bacteriophage therapy was the development of antibiotics. Today, the Western world has a renewed interest in bacteriophage therapy as a treatment to combat antibiotic-resistant strains of pathogenic bacteria, sometimes referred to as “superbugs.”
The History of the George Eliava Institute of Bacteriophage, Microbiology, and Virology
The Tbilisi Institute, founded by Professor George Eliava, opened its doors as a bacteriology laboratory in 1923. Professor Eliava met Felix d’Herelle in 1926 during a visit to the Pasteur Institute in Paris. After meeting at the Institute, d’Herelle and Eliava exchanged information about bacteriophage therapy. As a result of these exchanges, d’Herelle visited Eliava’s research laboratory at the Tbilisi Institute during 1934–1935. Josef Stalin (the dictator of the Soviet Union from roughly 1928 to 1953) invited d’Herelle to the Institute to investigate the possibility of bacteriophage therapy for military use. d’Herelle worked at Tbilisi Institute off and on for the year, hoping to form a great collaboration with Eliava. Unfortunately, the KGB executed Eliava in 1937.1 d’Herelle did not return to Tbilisi.
Even after Professor George Eliava’s death, the Tbilisi Institute continued bacteriology and bacteriophage research. In the 1940s, Institute researchers isolated bacteriophages to treat patients suffering from gas gangrene caused by Clostridium perfringens. The Tbilisi Institute merged with other research institutes and became the Institute of Vaccine and Sera in 1952. In 1988, the Institute was once again reorganized and renamed the George Eliava Research Institute of Bacteriophages and included such notable researchers as Zemphira Alavidze, Amiran Meipariani, and Revaz Adamia. The Institute of Vaccine and Sera manufactured bacteriophage sprays, salves, ointments, pills, and patches that were made available to the entire Soviet Union— especially the Soviet military, which was, and continues to be (now as the Russian Army), a major consumer of bacteriophage preparations. During the late 1980s, the Institute was at its peak in the production of bacteriophage products, employing 1,200 staff and manufacturing 2 tons of bacteriophage products every day. Bacteriophage therapy was and continues to be a routine part of treatments in clinics and hospitals in Eastern Europe. Bacteriophage preparations are sold at pharmacies for incredibly low prices and are available without a doctor’s prescription. In 1988, the Institute was rearranged and the scientific portion was renamed the George Eliava Research Institute of Bacteriophage.
The Institute facilities were damaged during the Georgian civil war (1991–1992). When the Soviet Union collapsed at the end of 1991, bacteriophage samples that had been identified and catalogued were lost. The bacteriophage collection dwindled from approximately 5,000 to 3,500. Electrical outages ruined many refrigerated bacteriophage libraries, and the Institute nearly closed. Privatization was implemented in order to save the collection. Dr. Leila Kalandarishvili led a team of Georgian scientists in acquiring the production division of the Eliava Institute. Dr. Kalandarishvili took over the financial responsibilities (she was the sole owner of this new company) required to maintain the production equipment, purchased needed electrical generators, and drilled a well in order to obtain a reliable water source. The bacteriophage collections were named Biomedic.
1 Eliava had become romantically involved with the same woman that the head of Stalin’s police was in love with. Reports out of the Soviet Union suggested that the KGB assassinated Eliava, denouncing him as an enemy of the people.
In 1994, Biomedic merged with Biopharm. Dr. Kalandarishvili was able to secure more than 20 Georgian patents on bacteriophage products, obtain the commercial licenses, and meet the Georgian government’s registration requirements. A 1997 BBC broadcast, The Virus That Cures, inspired energetic entrepreneurs from around the world to help save the Institute and its stocks.
In 2001, Dr. Kalandarishvili formed Advanced Biophage Technologies, LLC (ABT) in partnership with American businessman William B. McGall. Dr. Kalandarishvili and members of her scientific team continue to produce phage products, using the trade names of Pyophage, Intestiphage, Biosept, and other products for retail and over-the-counter sale.
At the time of this writing, many companies around the world are avidly pursuing bacteriophage therapy as an inexpensive, effective remedy to counter antibiotic resistance and hard-to-treat bacterial infections (see VIRUS FILE 20-1).
The American Phage Group
During the 1940s, scientists from universities throughout the United States who were studying bacteria and bacteriophages formed the “Phage Group.” Group members included luminaries such as Max Delbruck, Salvador Luria, and Alfred Hershey. These key members were educated as theoretical physicists or biologists, applying their approaches to the study of the physical nature of the gene. The Phage Group spent summers doing research experiments at Cold Spring Harbor Laboratory (located in Long Island, New York) and designed an annual course to improve laboratory instruction in the techniques of bacteriophage research.
Research at Cold Spring Harbor Laboratory centered on studying the basic biology of bacteriophages, including the optimization of one-step growth experiments. The development of bacteriophage plaque assays contributed to the foundations of modern animal virology (FIGURE 20-2). By 1952, the Phage Group consisted of about 50 members. Important problems of bacteriophage research were tackled because researchers focused on a select group of “authorized” phages, such as the Type (T) phages. During the 1950s Alfred Hershey and Martha Chase demonstrated that radioactively labeled phosphorous (present in DNA) entered bacterial host cells during T2 bacteriophage infection but that most of the radioactively labeled sulfur (present in proteins) did not. Delbruck, Luria, and Hershey shared the 1969 Nobel Prize in Physiology or Medicine for “their discoveries concerning the replication mechanism and the genetic structure of viruses.”

FIGURE 20-2 (a) Bacterial cells growing in log phase (left) and being lysed by bacteriophages (right). (b) Bacteriophage plaque assays from one-step growth experiments. The circular clearings present on the lawn of bacteria represent plaques, or regions where the bacteriophage infected the bacterium and lysed the cells. Each plaque arises from a single infectious bacteriophage. The infectious particle that gives rise to a plaque is called a PFu (plaque-forming unit). (c) Illustration showing the formation of plaques within a lawn of bacteria.
Phagoburn World Clinical Trials
PhagoBurn, a global collaborative project with the goal of evaluating phage therapy in serious burn victims, is in Phase I/II clinical trials (http://www.phagoburn.eu/). PhagoBurn is a European research and development (R&D) project funded by the European Commission. The 36-month project was launched on June 1, 2013, with actual trials beginning in July 2015. The project consists of 4 private and public partners and 11 clinical partners. The French company Les Laboratoires Brothier is providing Algosteril dressings for the clinical study. The private and public partners involved in PhagoBurn are:
Pherecydes Pharma
Clean Cells
Statitec
France Europe Innovation
The 11 clinical partners involved in PhagoBurn are:
The French Ministry of Defence through its Military Health Service and Percy Military Hospital (Belgium)
The Royal Military Academy of Belgium, through the Queen Astrid Military Hospital (Belgium)
Lausanne Burn Reference Centre (Switzerland)
Grand Hôpital de Charleroi–Loverval (Belgium)
CHU Liège (Belgium)
CHU Nantes (France)
Center Hospitalier Saint Joseph et Saint Luc (France)
CHU Bordeaux (France)
CHR Metz-Thionville (France)
Toulon Sainte-Anne Military Hospital (France)
Marseille Conception Hospital (France)
It is the first international clinical trial on phage therapy that meets international standards in clinical evaluation. The trial includes 220 burn patients divided into two groups: 110 patients who will be treated with bacteriophage cocktails that target Escherichia coli and a second group of 110 patients who will be treated with bacteriophage cocktails that target Pseudomonas aeruginosa.
20.2 Bacteriophage Ecology
Most of the bacteria that live in soil, sediment, and the open ocean are essential components of Earth’s micro-biota. Scientists at the University of Georgia (in Athens, Georgia) have estimated the total number of bacteria on Earth to be 4–6 × 1030 cells. The scientific community agrees that the global population of bacteriophages is about 1 × 1031. These values come from bacteriophage estimates in aquatic and soil environments. Less than 1% of the observed bacteriophages by electron microscopy can be cultivated in the laboratory. It is estimated there are about 108 genotypes. Bacteriophages may have the most genetic diversity of all viruses.
The marine viruses are the best characterized of the phage ecosystem. Marine bacteriophages infect and lyse bacteria in aquatic microbial communities. The lytic activity is recognized as the most important mechanism of recycling bacterial carbon in the marine environment. The dissolved organic carbon released by phage lysis of a bacterial population stimulates the growth of new bacterial populations that are part of the microbial food web in marine systems. Marine microbial ecology is a rapidly developing field.
20.3 The Biology of Bacteriophages: Composition and Structure
To date, over 5,400 bacteriophages have been examined by electron microscopy. The International Committee on the Taxonomy of Viruses (ICTV) recognizes 1 order, 13 families, and 31 genera of bacteriophages. Bacteriophages have four basic shapes or symmetries: binary (phages that have a head and tail structure), icosahedral (also referred to as cubic), helical (filamentous), and pleomorphic (FIGURE 20-3). All bacteriophages contain a head or capsid structure that varies in size or shape. The majority of bacteriophages contain a tail structure (FIGURE 20-4) that varies in size and length. The tail structure is a helical hollow tube. The genome of the bacteriophage passes through the tail and into the bacterial host during the initiation of infection. Binary bacteriophages have a genome length of 17–500 kilobases (kb) and tail lengths of 10–800 nm.
Three families contain bacteriophages that are enveloped. About 96% of all bacteriophages contain a double-stranded DNA (dsDNA) genome. The remaining 4% of bacteriophages contain genomes of single-stranded DNA (ssDNA), ssRNA, and dsRNA. Genomes code for as few as 3–5 gene products or as many as 100. The genomes of bacteriophages possess unusual or modified bases, protecting them from degradation by host nucleases during phage infection. The most famous group is the T-Type bacteriophages that are numbered T1 to T7. The most notorious bacteriophages are T4 and lambda (λ). Bacteriophage researchers have focused on the molecular biology of a handful of bacteriophages, including λ and T4. Not much is known about the molecular biology of the more than 5,000 other known bacteriophages. New bacteriophage families will likely be discovered in unusual habitats, such as hypersaline lagoons, volcanic hot springs, and cow rumens.

FIGURE 20-3 Diagram depicting the 13 different bacteriophage families grouped by type of nucleic acid genome. Tailed bacteriophages (depicted with the shaded background), are the most prevalent worldwide and infect eubacteria and the archeabacteria.

FIGURE 20-4 Images of tailed bacteriophages. (a) Enterobacter virus P2, Myoviridae family. (b) Bacillus licheniformis virus, Siphoviridae family. (c) Bacteriophage 3/K26 (infects Kurthia zopfii), Podoviridae family.
20.4 Overview of Bacteriophage Infection
Bacteriophages adsorb to surface receptors present on their bacterial host cells during the first step of the infection. Receptors may be host pili, proteins, oligosaccharides, or lipopolysaccharides (LPS). Adsorption is mediated by specialized structures such as tail fibers. The tailed T4 bacteriophage anchors its tail fibers onto the LPS receptors of its host. This causes a conformational change resulting in a contraction of the tail sheath and penetration of the bacterial membrane. The T4 DNA genome is subsequently injected into the bacterium through the tail tube (FIGURE 20-5).
Other bacteriophages may bind to a second receptor. Bacteriophages that do not have tails penetrate the host bacterium by producing polysaccharide-degrading enzymes that digest components of the bacterial cell wall. Bacteria develop resistance to bacteriophage infection when the host cell receptors are altered by a mutation. Losing some receptors offers no protection against infection by bacteriophages that bind to other host receptors. Researchers are very interested in engineering new receptor-recognition elements into the tail fibers of well-characterized bacteriophages so that they can infect taxonomically distant hosts.
The bacteriophage genome penetrates through the tail tube into the host cell. This is not an injection process, as it has often been portrayed. Bacteriophage T4 packages lysozyme in the base of its tail. Lysozyme is a virally encoded enzyme produced late during infection. It aids bacteriophages in escaping the host cell because it functions to degrade a portion of the peptidoglycan component of the bacterial cell wall, releasing mature bacteriophages. The DNA is drawn into the host cell by a process that is not well understood for most bacteriophages. Once inside the host cell, the bacteriophage DNA circularizes rapidly by means of sticky ends, or termini, or the linear ends are modified and protected from the bacterial nucleases.
As soon a bacteriophage DNA genome enters the cell, the host RNA polymerase recognizes the viral DNA promoters, resulting in transcription of the early genes. Phage gene expression is regulated temporally. In other words, specific genes are transcribed in a timely fashion. Translation of the early bacteriophage mRNAs is coupled with transcription. Examples of early proteins are repair enzymes (to repair the hole in the bacterial cell wall created during genomic penetration) and proteins that deactivate host proteases and block restriction enzymes (nucleases) that could degrade the bacteriophage DNA while copies of the bacteriophage genome are replicated. Subsequently, a set of late genes are transcribed into mRNAs and translated by the host protein synthesis machinery. Examples of late gene products are structural proteins that make up the capsomers of the head and tail of the bacteriophage and lysozyme, which is used by the bacteriophage to escape the host bacterium during a lytic infection. Progeny bacteriophages are released as the host bacterium is killed during its lysis. Lysozyme is packaged into the tail of some bacteriophages in order to initiate a new infection.

FIGURE 20-5 Bacteriophage T4 attaching to the ompC outer membrane protein receptor present on the outside surface of E. coli K-12 followed by the penetration of its genomic DNA into the cytoplasm of the bacterium.
How do the RNA bacteriophages regulate the amount of each protein synthesized during their replication? The secondary structure of the ssRNA phage genomes of MS2 and Q-beta regulates both the quantities of different bacteriophage proteins, but in addition it also operates temporal control of a switch in the ratios between the different proteins produced in infected host cells.
Lytic Infections: Assembly and Release
As soon as all bacteriophage “parts” are produced, new bacteriophages are assembled. A copy of the phage DNA genome is packaged, or “reeled into,” a preassembled icosahedral-shaped head. The tail and accessory structures (such as the base plate and tail fibers) are assembled, and a few molecules of lysozyme or a comparable enzyme are packaged into the tail base plate.
The remaining lysozyme or phage lysin, endolysins, muramidases, or virolysins inside of the infected bacterium allow the bacteriophage progeny to escape or to be released outside of the bacterium. These enzymes hydro-lyze specific bonds in the murein or peptidoglycan layer of the bacterial cell wall. The murein and peptidoglycan components of the cell wall are responsible for the rigidity and mechanical strength of bacterial cells. Another key enzyme, holin, is used by phages to create pores in the inner membrane of the host cell at the appropriate time to allow lysin or similar enzymes to reach the peptidoglycan, facilitating host lysis and bacteriophage release. Ultimately, the bacterial host cell is killed by cell lysis. This type of infection is referred to as a lytic infection. Lysis of the host cell is a tightly timed event (FIGURE 20-6).
Lysogenic (Temperate) Infections
Lysogenic, or temperate, bacteriophages infect their hosts, but instead of killing the host during a lytic infection their genome becomes integrated into a specific region of the host chromosome and the host cell remains alive. After penetration, the viral DNA integrates into the host chromosome and replicates every time the bacterial polymerase synthesizes its chromosomal DNA during cell division. Lysogenic cells divide, giving rise to lysogenic progeny. When the phage genome is integrated into the bacterial chromosome, it is called a prophage. Some temperate bacteriophages encode transposase, which allows the phage DNA genome to insert randomly into the bacterial chromosome. Other bacteriophage genomes are integrated into specific locations within the bacterial chromosome. During the prophage state, all transcription of the bacteriophage genes are repressed except for the mRNA that encodes a repressor protein. The repressor prevents the transcription and ultimately translation or synthesis of enzymes and proteins required for the lytic cycle.

FIGURE 20-6 Illustration of E. coli bacteriophage T4 lytic replication cycle. The phage attaches to a receptor on the outside of the host bacterium. Subsequently, phage DNA penetrates the bacterial cell wall (far right side of the bacterium) and enters the cytoplasm of the host cell where phage DNA replication takes place. The coupling of mRNA transcription and translation of phage proteins takes place before the final packaging of the phage DNA occurs within assembled heads. Tails are assembled and attached to the head in order to construct the progeny phages. Progeny phages are released through pores created by enzymes such as holins and the bacterium is killed as it is lysed. A single replication cycle is about 25 minutes long.
If the repressor becomes inactivated (loses function), phage DNA is excised from the bacterial chromosome. The excised DNA (bacteriophage genome) acts like a lytic phage that is able to produce new bacteriophages that are released during cell lysis. This spontaneous derepression, or induction, happens about once in every 10,000 cell divisions (FIGURE 20-7). Temperate bacteriophages carry host genes from one bacterial cell to another in a process called transduction.
20.5 Bacteriophages Create Pathogenic Bacteria in Nature
Bacteriophages may contribute to the virulence of pathogenic bacteria. Sometimes a prophage may carry genes that alter the phenotype of a bacterium in a process called lysogenic conversion. For example, the pathogen Corynebacterium diphtheriae produces a toxin responsible for diphtheria only if it carries the temperate bacteriophage called β. The tox gene is located near one end of the phage genome. Diphtheria is a very contagious and potentially life-threatening disease that usually attacks the mucous membranes of the throat and nose. The hallmark sign of diphtheria is a thick, gray covering in the back of the throat that can make breathing difficult. It is very rare today because of widespread vaccination. The diphtheria vaccine is produced by cultivating the C. diphtheriae that contains the β prophage in liquid medium. The bacterial culture is filtered and the filtrate is treated with formaldehyde, converting the β phage toxin to a toxoid. Single-antigen diphtheria toxoid is not available for vaccination. The diphtheria toxoid is combined with both the Clostridium tetani (bacterium that causes tetanus) toxoid and acellular pertussis vaccine as DTaP.
The infection of Streptococcus pyogenes with the temperate bacteriophage T12 results in the lysogenic conversion of a nontoxigenic strain to a bacterium that produces pyrogenic (also called erythrogenic) exotoxins. Pyrogenic exotoxins cause a fever along with the rash of scarlet fever that sometimes presents in individuals suffering from Streptococcus pyogenes infections that cause strep throat (FIGURE 20-8). The loss of the T12 prophage may render the bacterium nonpathogenic. The majority of bacteriophages possessing virulence genes are temperate phages that form stable lysogens. A lysogen is a bacterial cell that has been infected with a temperate bacteriophage (one that does not destroy the cell).

FIGURE 20-7 Replication cycle of a temperate bacteriophage. The DNA of temperate phages is incorporated into the host bacterial DNA where it is a prophage in a lysogenic replication cycle (right side of illustration) until an event occurs in which the prophage is excised from the host DNA and a lytic replication cycle is initiated by the bacteriophage (left side of illustration).
The evolution of toxigenic pathogens depended extensively on bacteriophage infections and exchanges of genetic information. Due to the lack of genetic and bacteriophage systems available to study the mechanisms of toxin gene transfer, scientists have barely scratched the surface in advancing knowledge of bacteriophage molecular biology and evolution. TABLE 20-1 lists examples of virulence genes carried by bacteriophages.
20.6 Control of Bacteriophages in Industrial Fermentation
Bacteria are used in a variety of food fermentation processes because they are able to break down complex organic substances into simpler ones. Examples of commercial food fermentation products are yogurt, cheese, sauerkraut, and soy sauce. The pharmaceutical and biotechnology industries use bacteria on a large scale to produce products such as alcohols, vitamins, amino acids, enzymes, hormones, biopolymers, antibiotics, and other therapeutics (FIGURE 20-9).

FIGURE 20-8 This child has the scarlet fever rash caused by a lysogenic Streptococcus pyogenes bacterium.
Table 20-1 Bacteriophage-Acquired Virulence Factors
Bacterial Origin of Virulence Factor | Bacteriophage | Toxin or Other Gene Product | Disease |
---|---|---|---|
Corynebacterium diphtheriae | β | Diphtheria toxin | Diphtheria |
Streptococcus pyogenes | T12 | Erythrogenic toxins | Scarlet fever |
Clostridium botulinum | Clostridial phages (e.g., CE β) | Botulinum toxin | Botulism (food poisoning) |
Vibrio cholerae | CTX | Cholera toxin | Cholera |
Escherichia coli | H-19B | Shiga-like toxins | Hemorrhagic diarrhea |
Staphylococcus aureus | Φ315, Φ13, ΦMu50A | Enterotoxins P and A | Food poisoning |
Mycoplasma arthritidis | MAV1 | Outer membrane protein (vir gene) | Arthritis involving two or more joints? |
Bacteriophages are ubiquitous in nature. Any bacterial strain can be infected by bacteriophages or harbor one or more prophages. All industrial fermentations that utilize bacteria to produce food or commodity chemicals are vulnerable to bacteriophage infections. Bacteriophage researcher Roger Hendrix provides us with the following analogy: “If a bacteriophage were the size of a cockroach—these cockroaches would cover the surface of the earth in a layer that is 31,071 miles or 50,000 km deep!” It is a nightmare statistic to laboratory staff working in a commercial fermentation factory, where bacteriophages really are a threat to production.

FIGURE 20-9 Bacterial fermentation vessel used to manufacture enzymes for medical and industrial use. This vessel can hold up to 120,000 liters of bacteria grown in liquid media.
Attacks by bacteriophages can cause serious problems in the bacterial fermentation production process and result in considerable economic losses. An entire fermentation batch may be lost due to phage contamination. Examples of common products and their bacterial hosts in fermentation-based industries are listed in TABLE 20-2. Approximately 1–10% of dairy product fermentation batches are lost to bacteriophage infection. This usually happens because the raw starting materials can be contaminated with undetectable numbers of bacteriophages.
Table 20-2 Common Products and Their Bacterial Hosts in Fermentation-Based Industries
Product | Bacterial Host |
---|---|
Yogurt | Lactobacillus delbrueckii, subspecies bulgaricus |
Streptococcus thermophiles | |
Cheese | Lactobacillus delbrueckii, subspecies lactis |
Lactobacillus helveticus | |
Lactobacillus lactis | |
Propionibacterium freudenreichii | |
Streptococcus thermophilus | |
Sourdough bread | Lactobacillus fermentum |
Sauerkraut | Leuconostoc mesenteroides |
Leuconostoc fallax | |
Lactobacillus plantarum | |
Vinegar | Acetobacter spp. |
Soy sauce | Tetragenococcus halophila |
Chloramphenicol | Streptomyces venezuelae |
Streptomycin | Streptomyces griseus |
Tetracycline | Streptomyces aureofaciens |
L-glutamic acid | Brevibacterium lactofermentum |
Insecticide (BT) | Bacillus thuringiensis |
Silage | Lactobacillus plantarum |
Hormones, enzymes, and other biotechnology products | Escherichia coli |
Butanol | Clostridium beijerinckii |
Acetone | Clostridium spp. |
Table 20-3 Bacteriophage Control Measures in the Dairy Food Industry
Rotating bacterial starter cultures Using starter cultures that do not contain strains of similar bacteriophage sensitivity patterns Installing air filtration (HEPA) systems Preparing starter cultures in a positively pressured room Cleaning and chlorinating vessels between fills Performing daily tests for bacteriophage detection Conducting regular inspections of equipment surfaces to remove biofilms Spraying the environment with adequate disinfecting agents Rotating manufacturing processes |
Laboratory staff at fermentation facilities should be trained to expect and respond to bacteriophage outbreaks, because the problem is unavoidable. Effective cleaning and monitoring procedures, especially during the bacteriophage “seasons” of January to March and October to November, is warranted. Measures used to control bacteriophages in the dairy industry are listed in TABLE 20-3. Dairy plants typically harbor 105 plaque-forming units per cubic meter of air. Effective control measures are needed to minimize bacteriophage contamination in industrial facilities that utilize bacteria to produce food products.
20.7 Biofilms and Bacteriophages
Bacteria can adhere to solid surfaces to form a slimy, slippery coat called a biofilm. Biofilms are found on most wet surfaces in the environment, including:
On river rocks
On the walls of limestone caves
In pipes
On industrial equipment
In the hulls of ships
On teeth, forming plaque
In the middle ear in patients with persistent otitis media (ear infections)
On the lining of the colon
On sutures
In the lungs of cystic fibrosis patients
On medical devices such as catheters
On stents
On heart valves and surrounding tissues of the heart
On orthopedic devices
On facial implants
In chronic wounds
Biofilms are complex, sessile (permanently attached) communities of bacteria that may consist of a single bacterial species or hundreds or even thousands of species. The biofilm bacteria produce extracellular polysaccha-ride polymers that surround and encase the microbes, facilitating their adhesion to surfaces (FIGURES 20-10 and 20-11). Biofilms can cause environmental problems or chronic bacterial infections in humans.
Development of bacterial biofilms has been intensely studied in vitro using confocal scanning laser microscopy to observe bacteria tagged with green fluorescent protein (GFP). For example, Pseudomonas aeruginosa produces a mature in vitro biofilm in 5–7 days. In these experiments, free-moving (or motile by means of flagellar movement) GFP-tagged bacteria reversibly attach to a surface that may be covered by a layer of proteins, such as a those found in a pellicle. At this stage, the bacteria are still susceptible to antibiotics. Subsequently, the bacteria irreversibly bind to the surface within the next several hours and multiply, forming microcolonies on the surface. During microcolony formation, the bacteria produce the extracellular polysaccharide (EPS) polymers around the microcolonies. In vitro, the biofilm forms a mushroom-like or tower-like structure in the mature biofilm. Quorum sensing occurs in the microcolonies and mature biofilm. Quorum sensing is a form of bacterial cell-to-cell communication. Bacteria use quorum sensing to regulate gene expression in response to fluctuations in population density. Once microcolonies are formed, the biofilm has maximum tolerance (resistance) to antibiotic therapy. Biofilm-growing bacteria may require up to 100- to 1,000-fold higher minimal inhibitory concentrations of antibiotics compared to the free-moving bacteria.

FIGURE 20-10 Electron micrograph of Staphylococcus aureus bacteria found on the surface of a patient’s catheter. The sticky-looking substance woven between the bacteria is composed of polysaccharides and is part of the biofilm that protects the bacteria from the penetration of antibiotics. Magnified 2,363×.

FIGURE 20-11 Scanning electron micrograph of a biofilm on the bristles of a used toothbrush.

FIGURE 20-12 Illustration depicting the formation of a biofilm based on in vitro experiments. Note: QS, quorum sensing; EPS, extracellular polysaccharide.
The mature biofilm can vary in thickness (25–100 μm), depending on the equilibrium bacterial growth and detachment of bacteria. Following this stage, distinct areas of the biofilm dissolve and the bacteria are detached and free to move to another location, where new biofilms are formed. The liberation process may be caused by bacteriophage activity within the biofilm (FIGURE 20-12).
Biofilms present an important challenge to patient medical care. Bacteria commonly isolated from medical devices are Enterococcus faecalis, S. aureus, S. epidermidis, Streptococcus viridans, E. coli, Klebsiella pneumoniae, Proteus mirabilis, and Pseudomonas aeruginosa. These bacteria originate from either the skin of the patient or healthcare workers, tap water (e.g., when entry ports of catheters are exposed to tap water), or other sources in the environment. Biofilms are tenacious and highly resistant to antimicrobial treatment, making infections difficult or impossible to treat.
The following strategies are used to prevent and treat biofilms on medical implants:
Using silver-impregnated catheters and cuffs
Incorporating inline filters into catheters
Coating devices with antibiotics
Disinfecting the surface site
Interest is growing in the role of bacteriophages in disrupting or reducing the formation of biofilms on medical implants. Studies by Curtin and Donlan attempting to pretreat catheter surfaces with bacteriophages to control biofilms are promising (FIGURE 20-13). Further research in this area is needed, particularly as the problem of antibiotic resistance continues to grow and traditional antibiotic treatments become less effective.
20.8 FDA-Approved Listeria-Specific Bacteriophage Preparations
Hot dogs and luncheon meats, as well as soft cheeses, such as feta, Brie, and Camembert (creamy milk cheese); blue-veined cheeses; or Mexican-style cheeses, such as queso blanco, that are not made with pasteurized milk may contain Listeria monocytogenes. Luncheon meats are especially prone to contamination with L. monocytogenes during slicing and packaging at food-processing plants. L. monocytogenes is a foodborne pathogen that is harmless in most healthy people but can cause listeriosis in pregnant women, newborns, and individuals with weakened immune systems.
Listeriosis during pregnancy can cause miscarriage or stillbirth, premature delivery, or infection of the newborn. Listeriosis has flulike symptoms, such as nausea and diarrhea. If the bacterial infection spreads to the nervous system, individuals experience a headache, stiff neck, confusion, loss of balance, and possibly convulsions. Listeriosis is 30% fatal in high-risk groups.

FIGURE 20-13 Illustrations showing applications of bacteriophages to treat biofilms. (a) Phages are embedded into a hydrogel present on a catheter as a pretreatment before the catheter is inserted into a patient. The bacteriophages should be able to infect and lyse bacterial cells, preventing the formation of biofilms on the catheter. (b) Phages are used to treat an existing biofilm present on a catheter, resulting in the hydrolysis of the extracellular polysaccharide polymers around the bacteria within the biofilm, liberating bacteria from the catheter, making them more susceptible to antibiotic and phage therapy.
In 2006, the FDA recognized the use of a cocktail of six bacteriophages in the form of an application spray as GRAS (Generally Recognized as Safe). The product is marketed as LISTEX P100 and is produced by the Phage Technology Center in the Netherlands.
LISTEX is used in the clean rooms of cheese, meat, and poultry processing plants to inhibit the growth of Listeria on products such as luncheon meats and hot dogs. The phage preparation is not declared as an ingredient on the label of a treated product. Additional products are being developed to reduce Campylobacter on chicken and Salmonella on fish, cheese, and meats.
Summary
No virology textbook should be without a chapter on bacteriophages. Bacteriophages—viruses that infect bacteria—have been an attractive model for scientists to learn about the basic biology of life. Bacteriophages are inexpensive and easy to work with in the laboratory. They have small genomes and reproduce rapidly, making them amenable to obtaining mutants that can be identified by rapid screening techniques.
The field of bacteriophage biology began during what is sometimes called the golden age of microbiology (~1870–1920) and then languished for decades. Today, applications using bacteriophages to combat infections caused by antibiotic-resistant bacteria and decontamination of disease-causing bacteria present on certain food products is once again thriving. Phage ecology is a rapidly developing field. Over 5,400 bacteriophages have been characterized by electron microscopy. The majority of bacteriophages are binary, in that they contain head and tail structures. About 96% of bacteriophages contain dsDNA genomes, and the remaining 4% contain ssDNA, ssRNA, or dsRNA genomes. Bacteriophages undergo a lytic or lysogenic infection. The host is killed (lysed) during a lytic infection and progeny phages are released. Lysogenic, or temperate, bacteriophages infect their bacterial hosts, but instead of killing the host their genome becomes integrated into a specific region of the bacterial chromo-some. The integrated bacteriophage genome is called a prophage. A prophage may carry genes that alter the phenotype of its host in a process called lysogenic conversion. The evolution of several toxigenic pathogens depended extensively on bacteriophage infections and exchanges of DNA. Examples include C. diphtheriae (causes diphtheria), S. pyogenes (causes strep throat and scarlet fever), and C. botulinum (causes food poisoning or botulism).
Felix d’Herelle is credited with discovering bacteriophages and the use of bacteriophage therapy to treat bacterial infections. He played a significant role in the establishment of the George Eliava Institute of Bacteriophage, Microbiology and Virology in Tbilisi in the Republic of Georgia. Bacteriophage therapy was used in the Soviet Union beginning in the 1920s. Bacteriophage sprays, salves, ointments, patches, and pills are still prepared and used in clinics and hospitals in the former Soviet Union and Eastern Europe. Today, bacteriophage therapy is being investigated by Western scientists as a potential therapy to defeat multidrug-resistant bacteria. Other applications of bacteriophages are to eliminate superbugs that form biofilms present on implanted medical devices and to treat ready-to-eat meats, fish, poultry, and soft cheeses with bacteriophages in order to eliminate foodborne pathogens such as L. monocytogenes, Salmonella spp., and Campylobacter spp. The first world industry-standard clinical trial is in progress. PhagoBurn is a collaborative project involving private, public, and clinical partners to study burn victims undergoing bacteriophage therapy.
Bacteriophages are ubiquitous in nature and can also threaten the food fermentation, biotechnology, and pharmaceutical industries that use bacteria on a large scale to produce fermented foods, chemicals, enzymes, hormones, biopolymers, antibiotics, and therapeutics. Effective control measures are needed to minimize bacteriophage contamination during the large-scale production of bacteria in these industries.
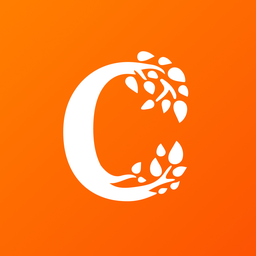
Full access? Get Clinical Tree
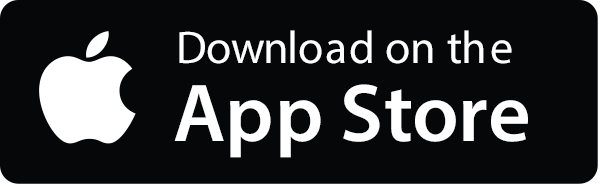
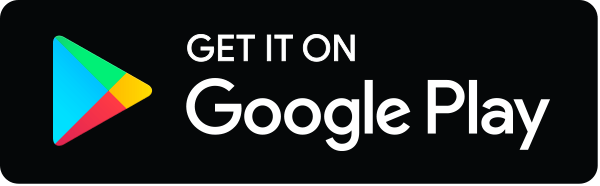