DNA synthesis occurs by the process of replication. During replication, each of the two parental strands of DNA serves as a template for the synthesis of a complementary strand. Thus, each DNA molecule generated by the replication process contains one intact parental strand and one newly synthesized strand (Fig. 12.1). In eukaryotes, DNA replication occurs during the S phase of the cell cycle, which is followed by the G2 phase. The cell divides during the next phase (M), and each daughter cell receives an exact copy of the DNA of the parent cell.
FIGURE 12.1 A replicating DNA helix. The parental strands separate at the replication fork. Each parental strand serves as a template for the synthesis of a new strand.
The Replication Fork. In both prokaryotes and eukaryotes, the site at which replication is occurring at any given moment is called the replication fork. As replication proceeds, the two parental strands separate in front of the fork. Behind the fork, each newly synthesized strand of DNA base-pairs with its complementary parental template strand. A complex of proteins is involved in replication. Helicases and topoisomerases unwind the parental strands, and single-strand binding proteins prevent them from reannealing.
The major enzyme involved in replication is a DNA polymerase that copies each parental template strand in the 3′-to-5′ direction, producing new strands in a 5′-to-3′ direction. Deoxyribonucleoside triphosphates serve as the precursors. One strand of newly synthesized DNA grows continuously, whereas the other strand is synthesized discontinuously in short segments known as Okazaki fragments. These fragments are subsequently joined by DNA ligase.
Initiation. DNA polymerase cannot initiate the synthesis of new strands. Therefore, a short primer is produced, which contains ribonucleotides (RNA). DNA polymerase can add deoxyribonucleotides to the 3′-end of this primer. This RNA primer is subsequently removed and replaced by deoxyribonucleotides.
Telomeres. The ends of linear chromosomes are called telomeres. The enzyme telomerase, an RNA-dependent DNA polymerase that carries its own RNA template, is required for their replication.
Errors and Repair. Errors that occur during replication can lead to deleterious mutations. However, many errors are corrected by enzyme activities associated with the complex at the replication fork. The error rate is thus kept at a very low level.
Damage to DNA molecules also causes mutations. Repair mechanisms correct DNA damage, usually by removing and replacing the damaged region. The intact, undamaged strand serves as a template for the DNA polymerase involved in the repair process.
Recombination. Although cells have mechanisms to correct replication errors and to repair DNA damage, some genetic change is desirable. It produces new proteins or variations of proteins that may increase the survival rate of the species. Genetic change is produced by unrepaired mutations and by a mechanism known as recombination, in which portions of chromosomes are exchanged. Recombination can also be used to repair double-strand breaks (DSBs) in DNA.
THE WAITING ROOM 
Like many patients, Isabel S. is finding it difficult to comply with her treatment regimen, which includes daily medications. She often forgets to take her pills. When she returns for a checkup, she asks if it is that important to take her pills every day for treatment of HIV.
Dianne A. responded to treatment for her diabetes mellitus but subsequently developed a low-grade fever, an increase in urinary urgency and frequency, and burning at the urethral opening with urination (dysuria). A urinalysis showed a large number of white blood cells and many gram-negative bacilli. A urine culture indicated many colonies of Escherichia coli, which is sensitive to several antibiotics, including the quinolone ciprofloxacin.
Calvin A. is a 46-year-old man who noted a superficial, brownish-black, 5-mm nodule with irregular borders in the skin on his chest. He was scheduled for outpatient surgery, at which time a wide excision biopsy was performed such that the complete mole is removed and biopsied. Examination of the nodule revealed histological changes characteristic of a malignant melanoma reaching a thickness of only 0.7 mm (Stage I).
Michael T. is a 62-year-old electrician who has smoked two packs of cigarettes a day for 40 years. He recently noted that his chronic cough had gotten worse. His physician ordered a chest radiograph, which showed a 2-cm nodule in the upper lobe of the right lung. A computed tomography (CT) scan was performed and confirmed a 2-cm nodule very concerning for malignancy. The patient was taken for surgery and the nodule excised. The pathology showed a poorly differentiated adenocarcinoma of the lung. Malignant neoplasms (new growth, a tumor) of epithelial cell origin (including the intestinal lining, cells of the skin, and cells lining the airways of the lungs) are called carcinomas. If the cancer grows in a gland-like pattern, it is an adenocarcinoma.
I. DNA Synthesis in Prokaryotes
The basic features of the mechanism of DNA replication are illustrated by the processes occurring in the bacterium E. coli. This bacillus grows symbiotically in the human colon. It has been extensively studied and serves as a model for the more complex, and consequently less well understood, processes that occur in eukaryotic cells.
A. Bidirectional Replication
Replication of the circular double-stranded DNA of the chromosome of E. coli begins with the binding of approximately 30 molecules of the protein DnaA at a single point of origin (known as the origin of replication), designated oriC, where the DNA coils around the DnaA core (Fig. 12.2). With the assistance of other proteins (e.g., a helicase, gyrase, and single-stranded binding protein), the two parental strands separate within this region, and both strands are copied simultaneously. Synthesis begins at the origin and occurs at two replication forks that move away from the origin bidirectionally (in both directions at the same time). The complex of proteins required for DNA replication is known as the replisome. Each replication fork contains its own replisome. Replication ends on the other side of the chromosome at a termination point. One round of synthesis, involving the incorporation of over 4 million nucleotides in each new strand of DNA, is completed in approximately 40 minutes. However, a second round of synthesis can begin at the origin before the first round is finished. These multiple initiations of replication allow bacterial multiplication to occur much more quickly than the time it takes to complete a single round of replication.
FIGURE 12.2 Bidirectional replication of a circular chromosome. Replication begins at the point of origin (oriC) and proceeds in both directions at the same time. Parental strands are shown in blue; newly synthesized strands are shown in red.
B. Semiconservative Replication
Each daughter chromosome contains one of the parental DNA strands and one newly synthesized, complementary strand. Therefore, replication is said to be semiconservative; that is, the parental strands are conserved but are no longer together. Each one is paired with a newly synthesized strand (see Figs. 12.1 and 12.2).
C. DNA Unwinding
Replication requires separation of the parental DNA strands and unwinding of the helix ahead of the replication fork. Helicases (an example of which is the protein DnaB) separate the DNA strands and unwind the parental duplex. Single-strand binding proteins prevent the strands from reassociating and protect them from enzymes that cleave single-stranded DNA (Fig. 12.3). Topoisomerases, enzymes that can break phosphodiester bonds and rejoin them, relieve the supercoiling of the parental duplex caused by unwinding. The protein DNA gyrase is a major topoisomerase in bacterial cells.
FIGURE 12.3 Proteins involved in separating and unwinding parental DNA strands at the replication fork in prokaryotes.
D. DNA Polymerase Action
Enzymes that catalyze the synthesis of DNA are known as DNA polymerases. E. coli has three DNA polymerases: Pol I, Pol II, and Pol III. Pol III is the major replicative enzyme (Table 12.1). All DNA polymerases that have been studied copy a DNA template strand in its 3′-to-5′ direction, producing a new strand in the 5′-to-3′ direction (Fig. 12.4). Deoxyribonucleoside triphosphates (dATP, dGTP, dCTP, and dTTP) serve as substrates for the addition of nucleotides to the growing chain.
TABLE 12.1 Functions of Bacterial DNA Polymerases
POLYMERASES | FUNCTIONSa | EXONUCLEASE ACTIVITYb |
Pol I | Filling of gap after removal of RNA primer | 5′-to-3′ and 3′-to-5′ |
DNA repair | ||
Removal of RNA primer in conjunction with RNase H | ||
Pol II | DNA repair | 3′-to-5′ |
Pol III | Replication; synthesis of DNA | 3′-to-5′ |
aSynthesis of new DNA strands always occurs 5′-to-3′.
bExonucleases remove nucleotides from the ends of DNA strands, acting either at the 5′-end (cleaving 5′-to-3′) or at the 3′-end (cleaving 3′-to-5′). Endonucleases cleave bonds within polynucleotide chains.
FIGURE 12.4 Action of DNA polymerase. Deoxyribonucleoside triphosphates serve as precursors (substrates) used by DNA polymerase to lengthen the DNA chain. DNA polymerase copies the DNA template strand in the 3′-to-5′ direction. The new strand grows 5′-to-3′. dGTP, deoxyguanosine triphosphate.
The incoming nucleotide forms a base pair with its complementary nucleotide on the template strand. Then, an ester bond is formed between the first (or α) 5′-phosphate of the incoming nucleotide and the free 3′-hydroxyl group at the end of the growing chain. Pyrophosphate is released. The release of pyrophosphate (formed from the β- and γ-phosphates of the nucleotide) and its subsequent cleavage by a pyrophosphatase provide the energy that drives the polymerization reaction.
DNA polymerases that catalyze the synthesis of new strands during replication exhibit a feature called processivity. They remain bound to the parental template strand while continuing to “process” down the chain rather than dissociating and reassociating as each nucleotide is added. Consequently, synthesis is much more rapid than it would be with an enzyme that was not processive.
E. Base-Pairing Errors
In E. coli, the replicative enzyme Pol III also performs a proofreading or editing function. This enzyme has 3′-to-5′-exonuclease activity in addition to its polymerase activity (see Table 12.1). If the nucleotide at the end of the growing chain is incorrectly base-paired with the template strand, Pol III removes this nucleotide before continuing to lengthen the growing chain. This proofreading activity eliminates most base-pairing errors as they occur. Only about one base pair in a million is mismatched in the final DNA product; the error rate is about 10−6. If this proofreading activity is experimentally removed from the enzyme, the error rate increases to about 10−3.
After replication, other mechanisms replace mismatched bases that escaped proofreading so that the fidelity of DNA replication is very high. The two processes of proofreading and postreplication mismatch repair result in an overall error rate of about 10−10, that is, <1 mismatched base pair in 10 billion.
F. RNA Primer Requirement
DNA polymerase cannot initiate the synthesis of new strands; it requires the presence of a free 3′-OH group to function. Therefore, a primer is required to supply the free 3′-OH group. This primer is an RNA oligonucleotide. It is synthesized in a 5′-to-3′ direction by an RNA polymerase (primase) that copies the DNA template strand. DNA polymerase initially adds a deoxyribonucleotide to the 3′-hydroxyl group of the primer and then continues adding deoxyribonucleotides to the 3′-end of the growing strand (Fig. 12.5).
FIGURE 12.5 Synthesis of DNA at the replication fork. (See Fig. 12.6 for the ligation reaction.)
G. The Replication Fork
Both parental strands are copied at the same time in the direction of the replication fork, an observation that is difficult to reconcile with the known activity of DNA polymerase, which can produce chains only in a 5′-to-3′ direction. Because the parental strands run in opposite directions relative to each other, synthesis should occur in a 5′-to-3′ direction toward the fork on one template strand and in a 5′-to-3′ direction away from the fork on the other template strand.
Okazaki resolved this dilemma by showing that synthesis on one strand, called the leading strand, is continuous in the 5′-to-3′ direction toward the fork. The other strand, called the lagging strand, is synthesized discontinuously in short fragments (see Fig. 12.5). These fragments, named for Okazaki, are produced in a 5′-to-3′ direction (away from the fork), but then are joined together so that, overall, synthesis proceeds toward the replication fork.
H. DNA Ligase
As replication progresses, the RNA primers are removed from Okazaki fragments, probably by the combined action of DNA polymerase I (Pol I, using its 5′-to-3′ exonuclease activity) and RNase H, an enzyme that removes RNA from DNA:RNA hybrids. Pol I fills in the gaps produced by removal of the primers. Because DNA polymerases cannot join two polynucleotide chains together, an additional enzyme, DNA ligase, is required to perform this function. The 3′-hydroxyl group at the end of one fragment is ligated to the phosphate group at the 5′-end of the next fragment (Fig. 12.6).
FIGURE 12.6 Action of DNA ligase. Two polynucleotide chains, one with a free 3′-OH group and one with a free 5′-phosphate group, are joined by DNA ligase, which forms a phosphodiester bond.
II. DNA Synthesis in Eukaryotes
The process of replication in eukaryotes is similar to that in prokaryotes. Differences in the processes are related mainly to the vastly larger amount of DNA in eukaryotic cells (over 1,000 times the amount in E. coli) and the association of eukaryotic DNA with histones in nucleosomes. Enzymes with DNA polymerase, primase, ligase, helicase, and topoisomerase activity are all present in eukaryotes, although these enzymes differ in some respects from those of prokaryotes.
A. Eukaryotic Cell Cycle
The cell cycle of eukaryotes consists of four phases (Fig. 12.7). The first three phases (G1, S, and G2) constitute interphase. Cells spend most of their time in these three phases, carrying out their normal metabolic activities. The fourth phase is mitosis, the process of cell division. This phase is very brief.
FIGURE 12.7 Eukaryotic cell cycle. The times given for the length of each phase are for cells growing in culture. DNA content is expressed as 2N (diploid), and after DNA replication, as 4N (tetraploid).
The first phase of the cell cycle, G1 (the first “gap” phase), is the most variable in length. Late in G1, the cells prepare to duplicate their chromosomes (e.g., by producing nucleotide precursors). In the second or S phase, DNA replicates. Nucleosomes disassemble as the replication forks advance. Throughout S phase, the synthesis of histones and other proteins associated with DNA is markedly increased. The amount of DNA and histones both double and chromosomes are duplicated. Histones complex with DNA, and nucleosomes are formed very rapidly behind the advancing replication forks.
During the third phase of the cell cycle, G2 (the second “gap” phase), the cells prepare to divide and synthesize tubulin for the construction of the microtubules of the spindle apparatus. Finally, division occurs in the brief mitotic or M phase.
Following mitosis, some cells reenter G1, repeatedly going through the phases of the cell cycle and dividing. Other cells arrest in the cycle after mitosis, never to divide again, or they enter an extended G1 phase (sometimes called G0), in which they remain quiescent but metabolically active for long periods of time. Upon the appropriate signal, cells in G0 are stimulated to reenter the cycle and divide.
B. Points of Origin for Replication
In contrast to bacterial chromosomes (see Section I.A of this chapter), eukaryotic chromosomes have multiple points of origin at which replication begins. “Bubbles” appear at these points on the chromosomes. At each end of a bubble, a replication fork forms; thus, each bubble has two forks. DNA synthesis occurs at each of these forks, as illustrated in Figure 12.8. As the bubbles enlarge, they eventually merge, and replication is completed. Because eukaryotic chromosomes contain multiple points of origin of replication (and, thus, multiple replicons, or units of replication), duplication of such large chromosomes can occur within a few hours.
FIGURE 12.8 Replication of a eukaryotic chromosome. Synthesis is bidirectional from each point of origin (O) and semiconservative. Each daughter DNA helix contains one intact parental strand (blue line) and one newly synthesized strand (red line).
C. Eukaryotic DNA Polymerases
Sixteen different DNA polymerases have been identified in eukaryotic cells. Examples of some of these polymerases, and their properties, are shown in Table 12.2. Polymerases δ (Pol δ) and ε (Pol ε) are the major replicative enzymes. Pol α is also involved in replication. Polymerases δ and ε, as well as Pol α, appear to be involved in DNA repair. Pol γ is located in mitochondria and replicates the DNA of this organelle. Polymerases ζ, κ, η, and ι, which lack 5′-exonuclease activity, are used when DNA is damaged and are known as bypass polymerases because they can “bypass” the damaged area of DNA and continue replication.
TABLE 12.2 Functions of Some Eukaryotic DNA Polymerases
POLYMERASE | FUNCTIONSa | EXONUCLEASE ACTIVITY |
Pol α | Replication (in a complex with primase and aids in starting the primer) | None |
DNA repair | ||
Pol β | DNA repair exclusively | None |
Pol γ | DNA replication in mitochondria | 3′-to-5′ |
Pol δ | Replication (processive DNA synthesis on lagging strand)b | 3′-to-5′ |
DNA repair | ||
Pol ε | Replication (processive DNA synthesis on leading strand)b | 3′-to-5′ |
DNA repair | ||
Pol κ | DNA repair (bypass polymerase)c | None |
Pol η | DNA repair (bypass polymerase) | None |
Pol ξ | DNA repair (bypass polymerase) | None |
Pol ι | DNA repair (bypass polymerase) | None |
aSynthesis of new DNA strands always occurs 5′-to-3′.
bRecent evidence suggests that leading strand synthesis utilizes both Pol δ and ε.
cBypass (translesion) polymerases are able to “bypass” areas of DNA damage and continue DNA replication. Some enzymes are error free and insert the correct bases; other enzymes are error-prone and sometimes insert incorrect bases.
D. The Eukaryotic Replication Complex
Many proteins bind at or near the replication fork and participate in the process of duplicating DNA (Fig. 12.9 and Table 12.3). Polymerases δ and ε are the major replicative enzymes. However, before the DNA polymerases can act, a primase associated with polymerase α (Pol α) produces an RNA primer (~10 nucleotides in length). Then, Pol α adds about 20 deoxyribonucleotides to this RNA and dissociates from the template because of the low processivity of Pol α. Pol α also lacks proofreading activity (3′-to-5′ exonuclease activity). On the leading strand, Pol ε adds deoxyribonucleotides to this RNA–DNA primer, continuously producing this strand. Pol ε is a highly processive enzyme.
TABLE 12.3 Major Proteins Involved in Replication
DNA polymerases | Add nucleotides to a strand growing 5′-to-3′, copying a DNA template 3′-to-5′ |
Primase | Synthesizes RNA primers |
Helicases | Separate parental DNA strands, i.e., unwind the double helix |
Single-strand binding proteins | Prevent single strands of DNA from reassociating |
Topoisomerases | Relieve torsional strain on parental duplex caused by unwinding |
Enzymes that remove primers | RNase H hydrolyzes RNA of DNA–RNA hybrids |
Flap endonuclease 1 (FEN1) recognizes “flap” | |
(Unannealed portion of RNA) near 5′-end of primer and cleaves downstream in DNA region of primer; the flap is created by polymerase δ displacing the primer as the Okazaki fragment is synthesized | |
DNA ligase | Joins, by forming a phosphodiester bond, two adjacent DNA strands that are bound to the same template |
PCNA | Enhances processivity of the DNA polymerases; binds to many proteins present at the replication fork |
PCNA, proliferating cell nuclear antigen.
FIGURE 12.9 Replication complex in eukaryotes. The lagging strand is shown looped around the replication complex to demonstrate that all DNA synthesis is in the 5′-to-3′ direction. Single-strand binding proteins (not shown) are bound to the unpaired, single-stranded DNA. Other proteins also participate in this complex (see text).
The lagging strand is produced from a series of Okazaki fragments (see Fig. 12.5). Synthesis of each Okazaki fragment is initiated by pol α and its associated primase, as described previously. After Pol α dissociates, Pol δ adds deoxyribonucleotides to the primer, producing an Okazaki fragment. Pol δ stops synthesizing one fragment when it reaches the start of the previously synthesized Okazaki fragment (see Fig. 12.5). The primer of the previously synthesized Okazaki fragment is removed by flap endonuclease 1 (FEN1) and RNase H. The gap left by the primer is filled by Pol δ using the parental DNA strand as its template and the newly synthesized Okazaki fragment as its primer. DNA ligase subsequently joins the Okazaki fragments together (see Fig. 12.6). Okazaki fragments are much smaller in eukaryotes than in prokaryotes (about 200 nucleotides vs. 1,000 to 2,000). Because the size of eukaryotic Okazaki fragments is equivalent to the size of the DNA found in nucleosomes, it seems likely that one nucleosome at a time may release its DNA for replication.
Obviously, eukaryotic replication requires many proteins. The complexity of the fork and the fact that it is not completely understood limits the detail shown in Figure 12.9. One protein that is not shown in Figure 12.9 is proliferating cell nuclear antigen (PCNA), which is involved in organizing and orchestrating the replication process on both the leading and lagging strands. PCNA is often used clinically as a diagnostic marker for proliferating cells.
Additional activities that occur during replication include proofreading and DNA repair. Pols δ and ε, which are part of the replication complex, exhibit the 3′-to-5′ exonuclease activity required for proofreading. Enzymes that catalyze repair of mismatched bases are also present (see Section III.B.3). Consequently, eukaryotic replication occurs with high fidelity; approximately one mispairing occurs for every 109 to 1012 nucleotides incorporated into growing DNA chains.
E. Replication at the Ends of Chromosomes
Eukaryotic chromosomes are linear, and the ends of the chromosomes are called telomeres. As DNA replication approaches the end of the chromosome, a problem develops in the lagging strand (Fig. 12.10). Either primase cannot lay down a primer at the very end of the chromosome, or, after DNA replication is complete, the RNA at the end of the chromosome is degraded. Consequently, the newly synthesized strand is shorter at the 5′-end, and there is a 3′-overhang in the DNA strand being replicated. If the chromosome became shorter with each successive replication, genes would be lost. How is this problem solved?
FIGURE 12.10 The end replication problem in linear chromosomes. After replication and removal of the RNA primers, the telomeres have 3′-overhangs. When these molecules are replicated, chromosome shortening will result. The figure depicts a linear chromosome with one origin of replication. At the origin, two replication forks are generated, moving in opposite directions, labeled as Fork 1 and Fork 2. As Fork 1 moves to the right, the bottom strand is read in the 3′-to-5′ direction, which means it is the template for the leading strand. The newly synthesized DNA complementary to the upper strand at Fork 1 will be the lagging strand. Now consider Fork 2. As this replication fork moves to the left, the upper strand is read in the 3′-to-5′ direction, so the newly synthesized DNA complementary to this strand will be the leading strand. For this fork, the newly synthesized DNA complementary to the bottom strand will be the lagging strand. The overhangs result from degradation of the RNA primers at the 5′-ends of the lagging strand, resulting in a 3′-overhang.
The 3′-overhang is lengthened by the addition of nucleotides so that primase can bind and synthesize a primer for the complementary strand. Telomeres consist of a repeating sequence of bases (TTAGGG for humans), which may be repeated thousands of times. The enzyme telomerase contains both proteins and RNA and acts as an RNA-dependent DNA polymerase (just like reverse transcriptase). The RNA within telomerase contains the complementary copy of the repeating sequence in the telomeres and can base-pair with the existing 3′-overhang (Fig. 12.11). The polymerase activity of telomerase then uses the existing 3′-hydroxyl group of the overhang as a primer and its own RNA as a template and synthesizes new DNA that lengthens the 3′-end of the DNA strand. The telomerase moves down the DNA toward the new 3′-end and repeats the process many times. When the 3′-overhang is sufficiently long, primase binds, and synthesis of the complementary strand is initiated. Even after this lengthening process, there is still a 3′-overhang that forms a complicated structure with telomere-binding proteins to protect the ends of the chromosomes from damage and nuclease attack once they have been lengthened.
FIGURE 12.11 Telomerase action. The RNA present in telomerase base-pairs with the overhanging 3′-end of telomeres and extends it by acting as both a template and a reverse transcriptase. After copying a small number of repeats, the complex moves down to the 3′-end of the overhang and repeats the process.
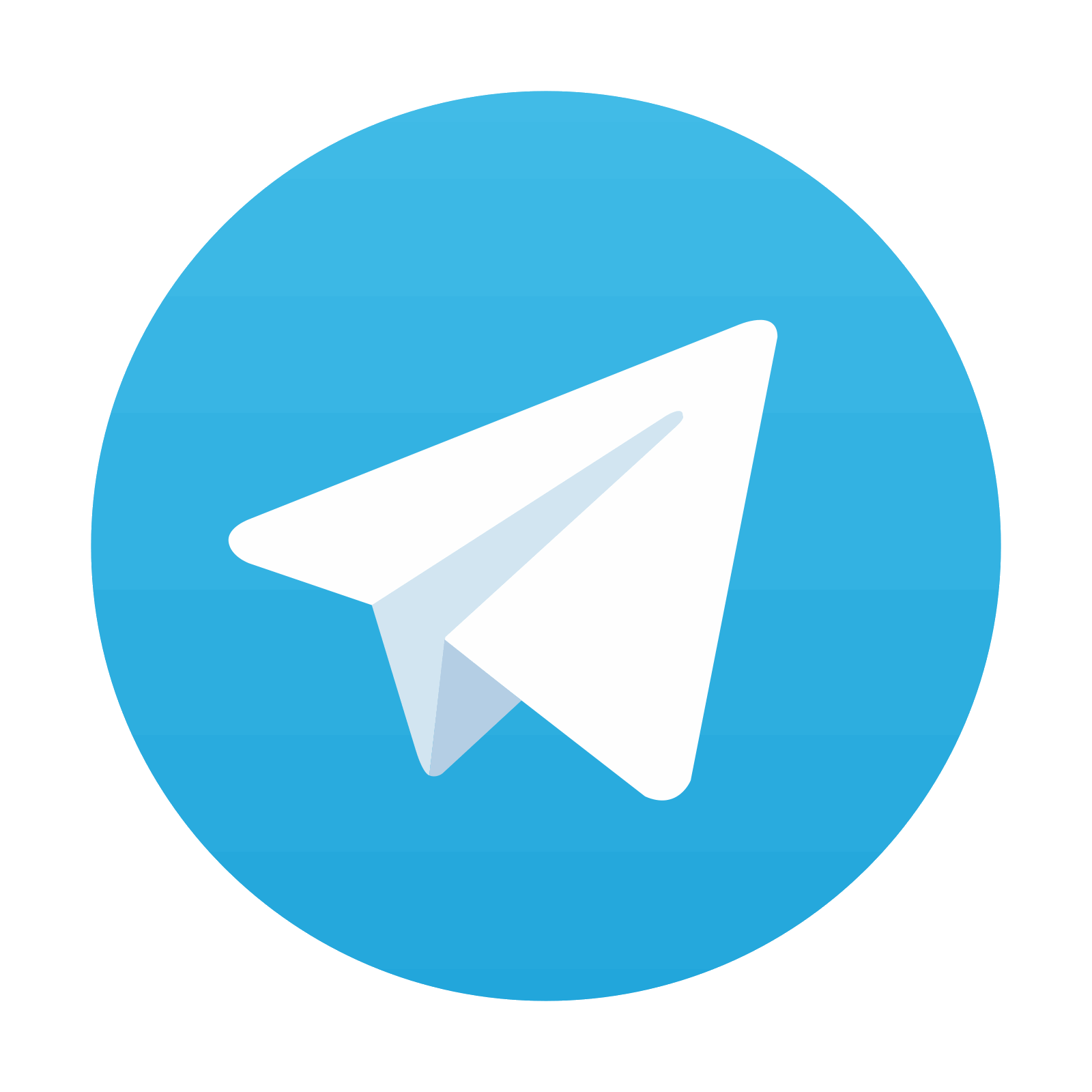
Stay updated, free articles. Join our Telegram channel
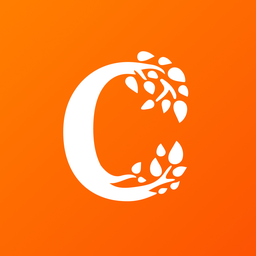
Full access? Get Clinical Tree
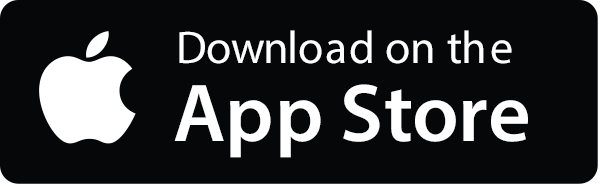
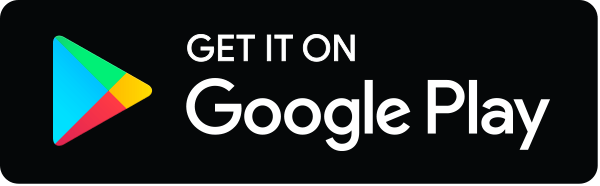