Because each of the 20 common amino acids has a unique structure, their metabolic pathways differ. Despite this, some generalities do apply to both the synthesis and degradation of all amino acids. These are summarized in the following sections. Because a number of the amino acid pathways are clinically relevant, we present most of the diverse pathways that occur in humans. However, we will be as succinct as possible.
Important Coenzymes. Pyridoxal phosphate (derived from vitamin B6) is the quintessential coenzyme of amino acid metabolism. In degradation, it is involved in the removal of amino groups, principally through transamination reactions and in donation of amino groups for various amino acid biosynthetic pathways. It is also required for certain reactions that involve the carbon skeleton of amino acids. Tetrahydrofolate (FH4) is a coenzyme that is used to transfer one-carbon groups at various oxidation states. FH4 is used in both amino acid degradation (e.g., serine and histidine) and biosynthesis (e.g., glycine). Tetrahydrobiopterin (BH4) is a cofactor that is required for ring hydroxylation reactions (e.g., phenylalanine to tyrosine).
Synthesis of the Amino Acids. Eleven of the 20 common amino acids can be synthesized in the body (Fig. 37.1). The other nine are considered “essential” and must be obtained from the diet. Almost all of the amino acids that can be synthesized by humans are amino acids used for the synthesis of additional nitrogen-containing compounds. Examples include glycine, which is used for porphyrin and purine synthesis; glutamate, which is required for neurotransmitter synthesis; and aspartate, which is required for both purine and pyrimidine biosynthesis.
FIGURE 37.1 Overview of the synthesis of the nonessential amino acids. The carbons of 10 amino acids may be produced from glucose through intermediates of glycolysis or the TCA cycle. The 11th nonessential amino acid, tyrosine, is synthesized by hydroxylation of the essential amino acid phenylalanine. Only the sulfur of cysteine comes from the essential amino acid methionine; its carbons and nitrogen come from serine. Transamination (TA) reactions involve pyridoxal phosphate (PLP) and another amino acid-α-keto acid pair. Acetyl CoA, acetyl coenzyme A; GDH, glutamate dehydrogenase.
Nine of the 11 “nonessential” amino acids can be produced from glucose plus, of course, a source of nitrogen, such as another amino acid or ammonia. The other two nonessential amino acids, tyrosine and cysteine, require an essential amino acid for their synthesis (phenylalanine for tyrosine, methionine for cysteine). The carbons for cysteine synthesis come from glucose; the methionine donates only the sulfur.
The carbon skeletons of the 10 nonessential amino acids derived from glucose are produced from intermediates of glycolysis and the tricarboxylic acid (TCA) cycle (see Fig. 37.1). Four amino acids (serine, glycine, cysteine, and alanine) are produced from glucose through components of the glycolytic pathway. TCA cycle intermediates (which can be produced from glucose) provide carbon for synthesis of the six remaining nonessential amino acids. α-Ketoglutarate is the precursor for the synthesis of glutamate, glutamine, proline, and arginine. Oxaloacetate provides carbon for the synthesis of aspartate and asparagine.
Regulation of the biosynthesis of individual amino acids can be quite complex, but the overriding feature is that the pathways are feedback-regulated such that as the concentration of free amino acid increases, a key biosynthetic enzyme is allosterically or transcriptionally inhibited. Amino acid levels, however, are always maintained at a level such that the aminoacyl-tRNA synthetases can remain active, and protein synthesis can continue.
Degradation of Amino Acids. The degradation pathways for amino acids are, in general, distinct from biosynthetic pathways. This allows for separate regulation of the anabolic and catabolic pathways. Because protein is a fuel, almost every amino acid has a degradative pathway that can generate NADH, which is used as an electron source for oxidative phosphorylation. However, the energy-generating pathway may involve direct oxidation, oxidation in the TCA cycle, conversion to glucose and then oxidation, or conversion to ketone bodies, which are then oxidized.
The fate of the carbons of the amino acids depends on the physiological state of the individual and the tissue in which the degradation occurs. For example, in the liver during fasting, the carbon skeletons of the amino acids produce glucose, ketone bodies, and CO2. In the fed state, the liver can convert intermediates of amino acid metabolism to glycogen and triacylglycerols. Thus, the fate of the carbons of the amino acids parallels that of glucose and fatty acids. The liver is the only tissue that has all of the pathways of amino acid synthesis and degradation.
As amino acids are degraded, their carbons are converted to (1) CO2, (2) compounds that produce glucose in the liver (pyruvate and the TCA cycle intermediates α-ketoglutarate, succinyl coenzyme A [succinyl CoA], fumarate, and oxaloacetate), and (3) ketone bodies or their precursors (acetoacetate and acetyl CoA) (Fig. 37.2). For simplicity, amino acids are considered to be glucogenic if their carbon skeletons can be converted to a precursor of glucose and ketogenic if their carbon skeletons can be converted directly to acetyl CoA or acetoacetate. Some amino acids contain carbons that produce a glucose precursor and other carbons that produce acetyl CoA or acetoacetate. These amino acids are both glucogenic and ketogenic.
FIGURE 37.2 Degradation of amino acids. A. Amino acids that produce pyruvate or intermediates of the TCA cycle. These amino acids are considered glucogenic because they can produce glucose in the liver. The fumarate group of amino acids produces cytoplasmic fumarate. Potential mechanisms whereby the cytoplasmic fumarate can be oxidized are presented in Section III.C.1. B. Amino acids that produce acetyl CoA or ketone bodies. These amino acids are considered ketogenic. HMG-CoA, hydroxymethylglutaryl coenzyme A; Methylmalonyl CoA, methylmalonyl coenzyme A; propionyl CoA, propionyl coenzyme A; Succinyl CoA, succinyl coenzyme A.
The amino acids that are synthesized from intermediates of glycolysis (serine, alanine, and cysteine) plus certain other amino acids (threonine, glycine, and tryptophan) produce pyruvate when they are degraded. The amino acids synthesized from TCA cycle intermediates (aspartate, asparagine, glutamate, glutamine, proline, and arginine) are reconverted to these intermediates during degradation. Histidine is converted to glutamate and then to the TCA cycle intermediate α-ketoglutarate. Methionine, threonine, valine, and isoleucine form succinyl CoA, and phenylalanine (after conversion to tyrosine) forms fumarate. Because pyruvate and the TCA cycle intermediates can produce glucose in the liver, these amino acids are glucogenic.
Some amino acids with carbons that produce glucose also contain other carbons that produce ketone bodies. Tryptophan, isoleucine, and threonine produce acetyl CoA, and phenylalanine and tyrosine produce acetoacetate. These amino acids are both glucogenic and ketogenic.
Two of the essential amino acids (lysine and leucine) are strictly ketogenic. They do not produce glucose, only acetoacetate and acetyl CoA.
THE WAITING ROOM 
Petria Y., a 4-month-old female infant, emigrated from the Ukraine with her French mother and Russian father 1 month ago. She was normal at birth but in the last several weeks was less than normally attentive to her surroundings. Her psychomotor development seemed to be delayed, and a tremor of her extremities had recently appeared. When her mother found her having gross twitching movements in her crib, she brought the infant to the hospital emergency room. A pediatrician examined Petria Y. and immediately noted a musty odor to the baby’s wet diaper. A drop of her blood was obtained from a heel prick and used to perform a Guthrie bacterial inhibition assay using a special type of filter paper. This screening procedure was positive for the presence of an excess of phenylalanine in Petria Y.’s blood.
Horace S., a 14-year-old boy, had a sudden weakness of the muscles on the left side of his face and of his left arm and leg. He was hospitalized with a tentative diagnosis of a cerebrovascular accident involving the right cerebral hemisphere.
Horace S.’s past medical history included a downward partial dislocation of the lenses of both eyes, for which he had had a surgical procedure. He also has a slight intellectual disability requiring placement in a special education group.
Horace S.’s left-sided neurological deficits cleared within 3 days, but a computerized axial tomogram (CT scan) showed changes consistent with a small infarct (a damaged area caused by a temporary or permanent loss of adequate arterial blood flow) in the right cerebral hemisphere. A neurologist noted that Horace S. had a slight waddling gait, which his mother said began several years earlier and was progressing with time. Further studies confirmed the decreased mineralization (decreased density) of the skeleton (called osteopenia if mild and osteoporosis if more severe) and high methionine and homocysteine but low cystine levels in the blood.
All of this information, plus the increased length of the long bones of Horace S.’s extremities and a slight curvature of his spine (scoliosis), caused his physician to suspect that Horace S. might have an inborn error of metabolism.
I. The Role of Cofactors in Amino Acid Metabolism
Amino acid metabolism requires the participation of three important cofactors. Pyridoxal phosphate (PLP) is the quintessential coenzyme of amino acid metabolism (Fig. 37.3). It is required for the following types of reactions involving amino acids: transamination, deamination, decarboxylation, β-elimination, racemization, and γ-elimination. Almost all pathways involving amino acid metabolism will require PLP at one step of the pathway.
FIGURE 37.3 Pyridoxal phosphate (PLP) attached covalently to an amino acid substrate. The arrows indicate which bonds are broken for the various types of reactions in which PLP is involved. X and Y represent chemical leaving groups that may be present on the amino acid (such as the hydroxyl group on serine or threonine).
The coenzyme tetrahydrofolate (FH4), which is derived from the vitamin folate, is required in certain amino acid pathways to either accept or donate a one-carbon group. The carbon can be in various states of oxidation. Chapter 38 describes the reactions of FH4 in much more detail.
The coenzyme tetrahydrobiopterin (BH4) is required for ring hydroxylations. The reactions involve molecular oxygen, and one atom of oxygen is incorporated into the product. The second is found in water (see Chapter 24). BH4 is important for the synthesis of tyrosine and neurotransmitters (see Chapter 46).
II. Amino Acids Derived from Intermediates of Glycolysis
Four amino acids are synthesized from intermediates of glycolysis: serine, glycine, cysteine, and alanine. Serine, which produces glycine and cysteine, is synthesized from 3-phosphoglycerate, and alanine is formed by transamination of pyruvate, the product of glycolysis (Fig. 37.4). When these amino acids are degraded, their carbon atoms are converted to pyruvate or to intermediates of the glycolytic/gluconeogenic pathway and, therefore, can produce glucose or be oxidized to CO2.
FIGURE 37.4 Amino acids derived from intermediates of glycolysis. These amino acids can be synthesized from glucose. Their carbons can be reconverted to glucose in the liver.
A. Serine
In the biosynthesis of serine from glucose, 3-phosphoglycerate is first oxidized to a 2-keto compound (3-phosphohydroxypyruvate), which is then transaminated to form phosphoserine (Fig. 37.5). Phosphoserine phosphatase removes the phosphate, forming serine. The major sites of serine synthesis are the liver and kidney.
FIGURE 37.5 The major pathway for serine synthesis from glucose is on the left, and for serine degradation on the right. Serine levels are maintained because serine causes repression (↓) of 3-phosphoglycerate dehydrogenase synthesis. Serine also inhibits (⊖) phosphoserine phosphatase. ADP, adenosine diphosphate; ATP, adenosine triphosphate; NAD, nicotinamide adenine dinucleotide; PEP, phosphoenolpyruvate; Pi, inorganic phosphate; PLP, pyridoxal phosphate.
Serine can be used by many tissues and is generally degraded by transamination to hydroxypyruvate, followed by reduction and phosphorylation to form 2-phosphoglycerate, an intermediate of glycolysis that forms phosphoenolpyruvate (PEP) and, subsequently, pyruvate. Serine also can undergo β-elimination of its hydroxyl group, catalyzed by serine dehydratase, to form pyruvate directly.
Regulatory mechanisms maintain serine levels in the body. When serine levels fall, serine synthesis is increased by induction of 3-phosphoglycerate dehydrogenase and by release of the feedback inhibition of phosphoserine phosphatase (caused by higher levels of serine). When serine levels rise, synthesis of serine decreases because synthesis of the dehydrogenase is repressed and the phosphatase is inhibited (see Fig. 37.5).
B. Glycine
Glycine can be synthesized from serine and, to a minor extent, threonine. The major route from serine is by a reversible reaction that involves FH4 and PLP (Fig. 37.6). Tetrahydrofolate is a coenzyme that transfers one-carbon groups at different levels of oxidation. It is derived from the vitamin folate and is discussed in more detail in Chapter 38. The minor pathway for glycine production involves threonine degradation (this is an aldolase-like reaction because threonine contains a hydroxyl group located two carbons from the carbonyl group).
FIGURE 37.6 Metabolism of glycine. Glycine can be synthesized from serine (major route) or threonine. Glycine forms serine or CO2 and NH4+ by reactions that require tetrahydrofolate (FH4). Glycine also forms glyoxylate, which is converted to oxalate or to CO2 and H2O. N5,N10–CH2–FH4, N5,N10-methylene tetrahydrofolate (see Chapter 38); NAD, nicotinamide adenine dinucleotide; PLP, pyridoxal phosphate; TPP, thiamine pyrophosphate.
The conversion of glycine to glyoxylate by the enzyme D-amino acid oxidase is a degradative pathway of glycine that is clinically relevant. Once glyoxylate is formed, it can be oxidized to oxalate, which is sparingly soluble and tends to precipitate in kidney tubules, leading to formation of kidney stones. Approximately 40% of oxalate formation in the liver comes from glycine metabolism. Dietary oxalate accumulation has been estimated to be a low contributor to excreted oxalate in the urine because of poor absorption of crystallized oxalate in the intestine.
Generation of energy from glycine occurs through a dehydrogenase (glycine cleavage enzyme) that oxidizes glycine to CO2, ammonia, and a carbon that is donated to FH4.
C. Cysteine
The carbons and nitrogen for cysteine synthesis are provided by serine, and the sulfur is provided by methionine (Fig. 37.7). Serine reacts with homocysteine (which is produced from methionine) to form cystathionine. This reaction is catalyzed by cystathionine β-synthase. Cleavage of cystathionine by cystathionase produces cysteine and α-ketobutyrate, which forms succinyl CoA via propionyl CoA. Both cystathionine β-synthase (β-elimination) and cystathionase (γ-elimination) require PLP.
FIGURE 37.7 Synthesis and degradation of cysteine. Cysteine is synthesized from the carbons and nitrogen of serine and the sulfur of homocysteine (which is derived from methionine). During the degradation of cysteine, the sulfur is converted to sulfate and either excreted in the urine or converted to 3′-phosphoadenosine 5′-phosphosulfate (PAPS, the universal sulfate donor), and the carbons are converted to pyruvate. ATP, adenosine triphosphate; Methylmalonyl CoA, methylmalonyl coenzyme A; propionyl CoA, propionyl coenzyme A; PLP, pyridoxal phosphate; Succinyl CoA, succinyl coenzyme A.
Cysteine inhibits cystathionine β-synthase and, therefore, regulates its own production to adjust for the dietary supply of cysteine. Because cysteine derives its sulfur from the essential amino acid methionine, cysteine becomes essential if the supply of methionine is inadequate for cysteine synthesis. Conversely, an adequate dietary source of cysteine “spares” methionine; that is, it decreases the amount that must be degraded to produce cysteine.
When cysteine is degraded, the nitrogen is converted to urea, the carbons to pyruvate, and the sulfur to sulfate, which has two potential fates (see Fig. 37.7; see also Chapter 40). Sulfate generation, in an aqueous medium, is essentially generating sulfuric acid, and both the acid and sulfate need to be disposed of in the urine. Sulfate is also used in most cells to generate an activated form of sulfate known as PAPS (3′-phosphoadenosine 5′-phosphosulfate), which is used as a sulfate donor in modifying carbohydrates or amino acids in various structures (glycosaminoglycans) and proteins in the body.
The conversion of methionine to homocysteine and homocysteine to cysteine is the major degradative route for these two amino acids. Because this is the only degradative route for homocysteine, vitamin B6 deficiency or congenital cystathionine β-synthase deficiency can result in homocystinemia, which is associated with cardiovascular disease.
D. Alanine
Alanine is produced from pyruvate by a transamination reaction catalyzed by alanine aminotransaminase (ALT) and may be converted back to pyruvate by a reversal of the same reaction (see Fig. 37.4). Alanine is the major gluconeogenic amino acid because it is produced in many tissues for the transport of nitrogen to the liver.
III. Amino Acids Related to TCA Cycle Intermediates
Two groups of amino acids are synthesized from TCA cycle intermediates: one group from α-ketoglutarate and one from oxaloacetate (see Fig. 37.1). During degradation, four groups of amino acids are converted to the TCA cycle intermediates α-ketoglutarate, oxaloacetate, succinyl CoA, and fumarate (see Fig. 37.2A).
A. Amino Acids Related through α-Ketoglutarate/Glutamate
1. Glutamate
The five carbons of glutamate are derived from α-ketoglutarate either by transamination or by the glutamate dehydrogenase reaction (see Chapter 36). Because α-ketoglutarate can be synthesized from glucose, all of the carbons of glutamate can be obtained from glucose (see Fig. 37.1). When glutamate is degraded, it is likewise converted back to α-ketoglutarate either by transamination or by glutamate dehydrogenase. In the liver, α-ketoglutarate leads to the formation of malate, which produces glucose via gluconeogenesis. Thus, glutamate can be derived from glucose and reconverted to glucose (Fig. 37.8).
FIGURE 37.8 Amino acids related through glutamate. These amino acids contain carbons that can be reconverted to glutamate, which can be converted to glucose in the liver. All of these amino acids except histidine can be synthesized from glucose.
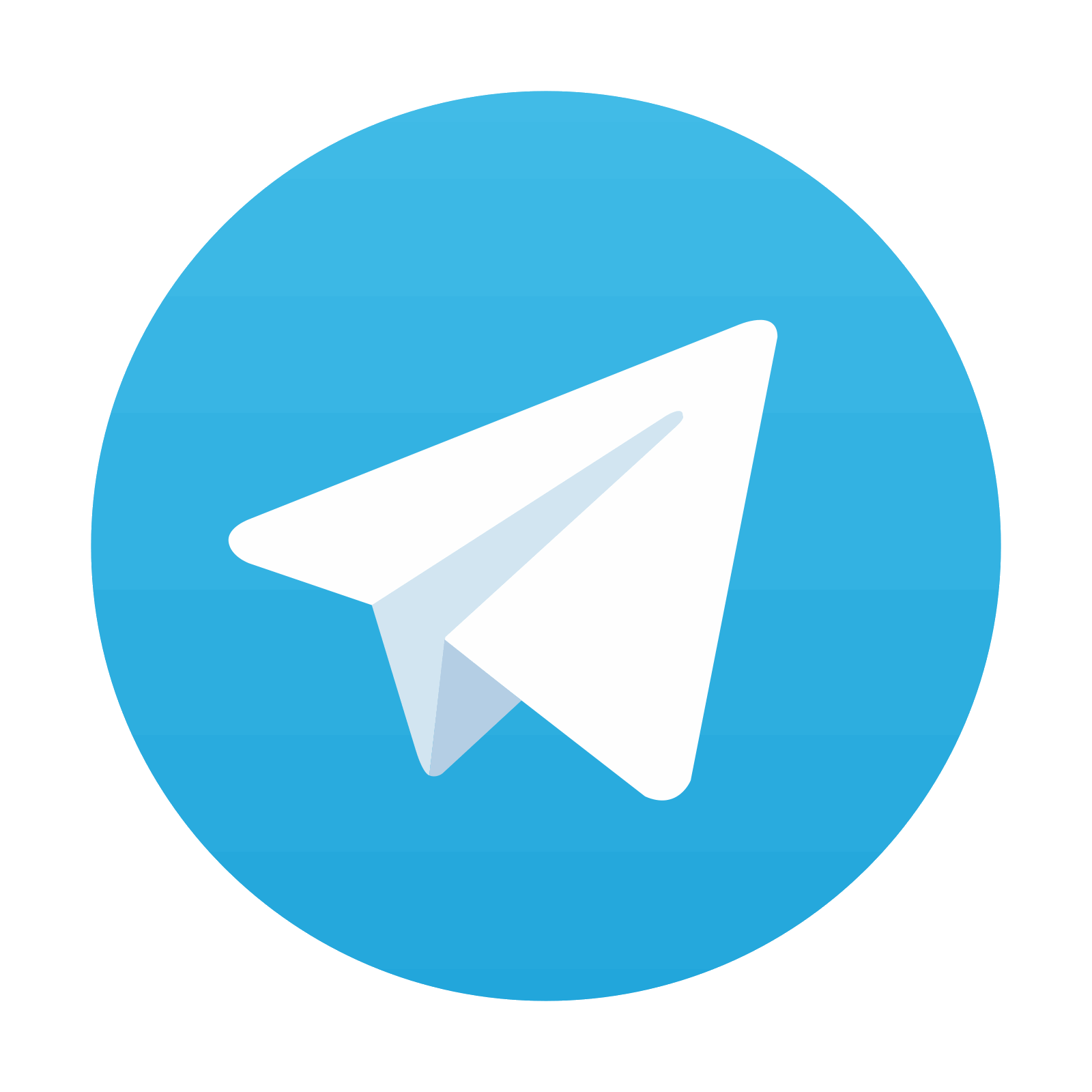
Stay updated, free articles. Join our Telegram channel
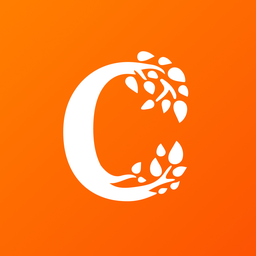
Full access? Get Clinical Tree
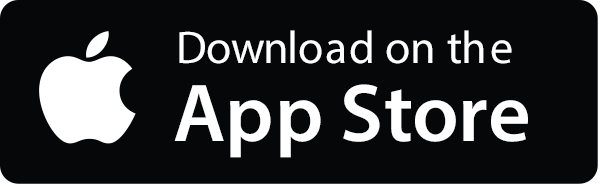
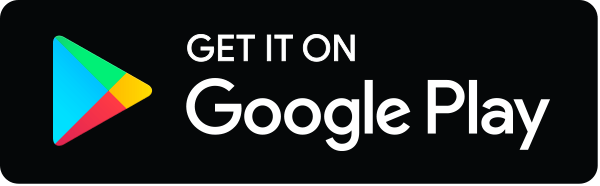