CHAPTER 6 Synaptic Transmission
Synaptic transmission is the major process by which electrical signals are transferred between cells within the nervous system (or between neurons and muscle cells or sensory receptors). Within the nervous system, synaptic transmission is usually conceived of as an interaction between two neurons that occurs in a point-to-point manner at specialized junctions called synapses. Two main classes of synapses are distinguished: electrical and chemical. However, as the list of chemical neurotransmitters has grown and as understanding of their mechanisms of action has increased, the definition and conception of what constitutes synaptic transmission has had to be refined and expanded. We no longer think of synaptic transmission as a process that involves only neurons, but now realize that glia form an important element of the synapse and that signaling occurs between neurons and glia. Moreover, in many cases neurotransmitter released at a synapse will act over a widespread territory rather than just at the synapse from which it is released. Thus, we must either generalize the definition of synaptic transmission or consider classically defined synaptic transmission as but one of several mechanisms by which cells in the nervous system communicate with each other. In this chapter we first describe the classic conception of synaptic transmission (electrical and chemical) and then introduce some of the nontraditional neurotransmitters and discuss how they have forced modifications in our conception of chemical communication between cells in the nervous system.
ELECTRICAL SYNAPSES
A gap junction is the morphological correlate of an electrical synapse (see also Chapter 1). These junctions are plaque-like structures in which the plasma membranes of coupled cells become closely apposed (the intercellular space narrows to approximately 3 nm) and filled with electron-dense material (Fig. 6-1). Freeze-fracture electron micrographs of gap junctions display regular arrays of intramembrane particles that correspond to proteins that form the intercellular channels connecting the cells. The typical channel diameter is large (1 to 2 nm), thus making it permeable not only to ions but also other small molecules up to approximately 1 kDa in size.
Electrical synapses are fast (essentially no synaptic delay) and bidirectional (i.e., current generated in either cell can flow across the gap junction to influence the other cell). In addition, they act as low-pass filters. That is, slow electrical events are much more readily transmitted than are fast signals such as action potentials. One important role for neuronal gap junctions appears to be synchronization of network activity. For example, the activity of inferior olivary neurons is normally synchronized but becomes uncorrelated when pharmacological blockers of gap junctions are injected into the inferior olive. It also appears that the patterns of electrical coupling by gap junctions may be highly specific. For example, neocortical interneurons almost exclusively couple to interneurons of the same type. This specific gap junction—coupling pattern suggests that multiple, independent, electrically coupled networks of interneurons may coexist across the neocortex.
CHEMICAL SYNAPSES
Unlike the situation at electrical synapses, at chemical synapses there is no direct communication between the cytoplasm of the two cells. Instead, the cell membranes are separated by a synaptic cleft of some 20 μm, and interaction between the cells occurs via chemical intermediaries known as neurotransmitters. Chemical synapses are generally unidirectional, and thus one can refer to the presynaptic and postsynaptic elements that are diagramed in Figure 6-2. The presynaptic element is often the terminal portion of an axon and is packed with small vesicles whose exact shape and size vary with the neurotransmitter that they contain. In addition, the presynaptic membrane apposed to the postsynaptic element has regions, known as active zones, of electron-dense material that corresponds to the proteins involved in transmitter release (Fig. 6-1, B). Moreover, mitochondria and rough endoplasmic reticulum are typically found in the presynaptic terminal. The postsynaptic membrane is also characterized by electron-dense material, which in this case corresponds to the receptors for the neurotransmitter.
Chemical synapses occur between different parts of neurons. Traditionally, focus has been placed on synapses formed by an axon onto the dendrites or soma of a second cell (axodendritic or axosomatic synapses), and our description will be based primarily on such synapses. However, there are many additional types of chemical synapses, such as axoaxonic (axon to axon), dendrodendritic (dendrite to dendrite), and dendrosomatic (dendrite to soma). Furthermore, complex synaptic arrangements are possible, such as mixed synapses, in which cells form both electrical and chemical synapses with each other; serial synapses, in which an axoaxonic synapse is made onto the axon terminal and influences the efficacy of that terminal’s synapse with yet a third element; and reciprocal synapses, in which both cells can release transmitter to influence the other. Figure 6-1, B, shows a complex synaptic arrangement, called a glomerulus, that involves both chemical and electrical synapses among the participating elements.
Much of what we know about chemical synapses comes from the study of two classic preparations, the frog neuromuscular junction (the synapse from a motor neuron onto the muscle) and the squid giant synapse (the synapse from a second-order neuron onto third-order neurons that innervate the muscle of the squid’s mantle; i.e., the motor neurons, which are the cells whose axons were used to characterize the conductances underlying the action potential [see Chapter 5]). The principles governing transmission at these synapses mostly apply to synapses within the mammalian CNS as well, at least with regard to synapses using what are called the “classic” neurotransmitters (see the section Neurotransmitters). Thus, much of the following discussion will be based on results from these two preparations; however, some differences in CNS synapses will also be pointed out.
Synaptic transmission at a chemical synapse may be summarized as follows. Synaptic transmission is initiated by arrival of the action potential at the presynaptic terminal. The action potential depolarizes the terminal, which causes Ca++ channels to open. The subsequent rise in [Ca++] within the terminal triggers the fusion of vesicles containing neurotransmitter with the plasma membrane. The transmitter is then expelled into the synaptic cleft, diffuses across it, and binds to specific receptors on the postsynaptic membrane. Binding of transmitter to receptors then causes the opening (or less often, the closing) of ion channels in the postsynaptic membrane, which in turn results in changes in the potential and resistance of the postsynaptic membrane that alter the excitability of the cell. The changes in membrane potential of the postsynaptic cell are termed excitatory and inhibitory postsynaptic potentials (EPSPs and IPSPs) (Fig. 6-3), depending on whether they increase or decrease, respectively, the cell’s excitability, which can be defined as its probability of firing action potentials. The transmitter acts for only a very short time (milliseconds) because reuptake and degradation mechanisms rapidly clear the transmitter from the synaptic cleft.
The succeeding sections will amplify specific points of this summary. However, it is worth mentioning at this point that some of the nonclassic types of neurotransmitters (e.g., neuropeptides and gaseous neurotransmitters such as nitric oxide) and the discovery of metabotropic receptors have required modifications of several aspects of this basic conception (a metabotropic receptor does not contain an ion channel but, instead, is coupled to a G protein that initiates second messenger cascades that can ultimately affect ion channels, whereas an ionotropic receptor contains the ion channel as an integral part of itself). Some of the differences between classic and peptide transmitters are listed in Table 6-1. More details on the properties of peptide and gaseous transmitters are provided in the relevant parts of the Neurotransmitters section of this chapter, and metabotropic receptors are covered in the Receptors section.
Table 6-1 Distinctions between Classic Nonpeptide Neurotransmitters and Peptide Neurotransmitters
Nonpeptide Transmitters | Peptide Transmitters |
---|---|
Synthesized and packaged in the nerve terminal | Synthesized and packaged in the cell body; transported to the nerve terminal by fast axonal transport |
Synthesized in active form | Active peptide formed when it is cleaved from a much larger polypeptide that contains several neuropeptides |
Usually present in small, clear vesicles | Usually present in large, electron-dense vesicles |
Released into a synaptic cleft | |
Action of many terminated because of uptake by presynaptic terminals via Na+-powered active transport | Action terminated by proteolysis or by the peptide diffusing away |
Typically, action has short latency and short duration (msec) | Action may have long latency and may persist for many seconds |
Calcium Entry Is the Signal for Transmitter Release
Depolarization of the presynaptic membrane by the action potential causes voltage-gated Ca++ channels to open, which makes it possible for Ca++ to flow into the terminal and trigger the release of transmitter. However, Ca++ will enter the terminal only if there is a favorable electrochemical gradient to do so. Recall that it is the combination of the concentration and voltage gradients that determines the direction of ion flow through open channels. Extracellular [Ca++] is high relative to intracellular [Ca++], which favors entry into the terminal; however, during the peak of the action potential, the membrane potential is positive, and the voltage gradient opposes the entry of Ca++ because of its positive charge. Thus, at the peak of the action potential, relatively little Ca++ enters the terminal because although the membrane is highly permeable to Ca++, the overall driving force is small. In fact, by using a voltage clamp, one can experimentally make the membrane potential positive and equal to the Nernst equilibrium potential for Ca++. If this is done, no Ca++ will enter the terminal despite Ca++ channels being open, and as a result no transmitter is released and no postsynaptic response is observed. This voltage is known as the suppression potential. If the membrane potential is rapidly made negative again (because of either the end of the action potential or by adjusting the voltage clamp), Ca++ rushes into the terminal as a result of the large driving force (which arises instantaneously on repolarization) and the high membrane permeability to Ca++ (which remains high because it takes the Ca++ channels several milliseconds to close in response to the new membrane potential), thereby resulting in release of transmitter and a postsynaptic response (Fig. 6-4).
Synaptic Vesicles and the Quantal Nature of Transmitter Release
How neurotransmitter is stored and how it is released are questions fundamental to synaptic transmission. Answering these questions began with two observations. The first was the discovery of small round or irregularly shaped organelles known as synaptic vesicles in presynaptic terminals by electron microscopy (Fig. 6-2). The second observation came from recordings of postsynaptic responses at the neuromuscular junction. Normally, an action potential in a motor neuron causes a large depolarization in the postsynaptic muscle, termed an end plate potential (EPP), which is equivalent to an EPSP in a neuron. However, under conditions of low extracellular [Ca++], the EPP amplitude is reduced (because the presynaptic Ca++ current is reduced, leading to a smaller rise in intracellular [Ca++], and transmitter release is proportional to [Ca++]). In this condition, the EPP is seen to fluctuate among discrete values (Fig. 6-5). Moreover, small, spontaneous depolarizations of the postsynaptic membrane, termed miniature end plate potentials (mEPPs), are observable. The amplitude of the mEPP (≤1 mV) corresponds to that of the smallest EPP evoked under low [Ca++], and the amplitudes of other EPPs were shown to be integral multiples of the mEPP amplitude; thus, it was natural to propose that each mEPP corresponded to the release of transmitter from a single vesicle and that EPPs represented the combined simultaneous release of transmitter from many vesicles.
Molecular Apparatus Underlying Vesicular Release
The small vesicles that contain nonpeptide neurotransmitters can fuse with the presynaptic membrane only at specific sites, called active zones. To become competent to fuse with the presynaptic membrane at an active zone, a small vesicle must first dock at the active zone. It must then undergo a priming process before the vesicle can fuse and release its transmitter into the synaptic cleft in response to an increase in local cytoplasmic [Ca++]. On the order of 25 proteins may play roles in docking, priming, and fusion. Some of these proteins are cytosolic, whereas others are proteins of the vesicle membrane or the presynaptic plasma membrane. The functions of most of these proteins are incompletely understood; however, knowledge of the molecular details of transmitter release has increased dramatically in recent years.
Synaptic Vesicles Are Recycled
There appear to be two distinct mechanisms by which vesicles are retrieved after release of their neurotransmitter content (Fig. 6-6). One mechanism is the endocytotic pathway commonly found in most cell types. Coated pits are formed in the plasma membrane, which then pinch off to form coated vesicles within the cytoplasm of the presynaptic terminal. These vesicles then lose their coat and undergo further transformations (i.e., acquire the correct complement of membrane proteins and be refilled with neurotransmitter) to become once again synaptic vesicles ready for release.
Recently, evidence for a second, more rapid recycling mechanism has been obtained (Fig. 6-6). It involves transient fusion of the vesicle to the synaptic membrane and has been called “kiss and run.” In this case, fusion of the vesicle with the synaptic membrane leads to the formation of a pore through which the transmitter is expelled, but there is no wholesale collapse of the vesicle into the membrane. Instead, the duration of the fusion is very brief, after which the vesicle detaches from the plasma membrane and reseals itself. Thus, the vesicle membrane retains its molecular identity. Its contents can then simply be replenished, thereby making the vesicle ready for use again.
Postsynaptic Potentials
where gx is the conductance of the channel to ion x, Vm is the membrane potential, and Ex is the Nernst equilibrium potential for ion x. In this case gx is similar for Na+ and K+, so the main determinant of net current is the relative driving forces (Vm − Ex). If the membrane is at its resting potential (typically around −70 mV), there is a strong driving force (Vm − ENa) for Na+ to enter the cell because this potential is far from the Na+ Nernst potential (about +55 mV), whereas there is only a small driving force for K+ to leave the cell because Vm is close to the K+ Nernst potential (about −90 mV). Thus, if acetylcholine-gated channels open when the membrane is at its resting potential, a large inward Na+ current and a small outward K+ current will flow through the acetylcholine channel, thereby resulting in a net inward current, which acts to depolarize the membrane.
The net inward current that results from opening such channels is called the excitatory postsynaptic current (EPSC). Figure 6-7, A, contrasts the time course of the EPSC and the resulting EPSP for fast synaptic transmission. The EPSC is much shorter (≈ 1 to 2 msec in duration) and corresponds to the time that the channels are actually open. The short duration of the EPSC is due to the fact that the released neurotransmitter remains in the synaptic cleft for only a short while before being either enzymatically degraded or taken up by either glia or the presynaptic terminal. Binding and unbinding of a neurotransmitter to its receptor take place rapidly, so once its concentration falls in the cleft, the postsynaptic receptor channels rapidly close as well and terminate the EPSC. Note how the end of the EPSC corresponds to the peak of the EPSP, which is followed by a long tail. The duration of the tail and the rate of the decay in EPSP amplitude reflect the passive membrane properties of the cell (i.e., its RC properties). In slow synaptic transmission, the duration of the EPSP reflects the activation and deactivation of biochemical processes more than the membrane properties. The long duration of even fast EPSPs is functionally important because it allows EPSPs to overlap and thereby summate. Such summation is central to the integrative properties of neurons (see the later section Synaptic Integration).
Normally, an EPSP depolarizes the membrane, and if this depolarization reaches threshold, an action potential is generated. However, consider what happens if the channels underlying the action potential are blocked and the membrane of the postsynaptic cell is experimentally depolarized by injecting current through an intracellular electrode. Because the membrane potential is now more positive, the driving force for Na+ is decreased and that for K+ increased. If the synapse is activated at this point, the net current through the receptor channel (the EPSC) will be smaller because of changes in the relative driving force. This implies that if the membrane potential is depolarized enough, there will be a point at which the Na+ and K+ currents through the channel are equal and opposite and thus there is no net current and no EPSP. If the membrane is depolarized beyond this point, there is a net outward current through the receptor channels, and the membrane will hyperpolarize (i.e., the EPSP will be negative). Thus, the potential at which there is no EPSP (or EPSC) is known as the reversal potential. For excitatory synapses, the reversal potential is usually around 0 mV (± 10 mV), depending on the synapse (Fig. 6-7, B and C).
IPSPs, like EPSPs, are triggered by the binding of neurotransmitter to receptors on the postsynaptic membrane and typically involve an increase in membrane permeability as a result of the opening of ligand-gated channels. They differ in that IPSP channels are permeable to only a single ionic species, either Cl− or K+. Thus, IPSPs will have a reversal potential equal to the Nernst potential of the ion carrying the underlying current. Typically, the Nernst potential for these ions is somewhat negative relative to the resting potential, so when IPSP channels open, there is an outward flow of current through them that results in hyperpolarization of the membrane (Fig. 6-3).
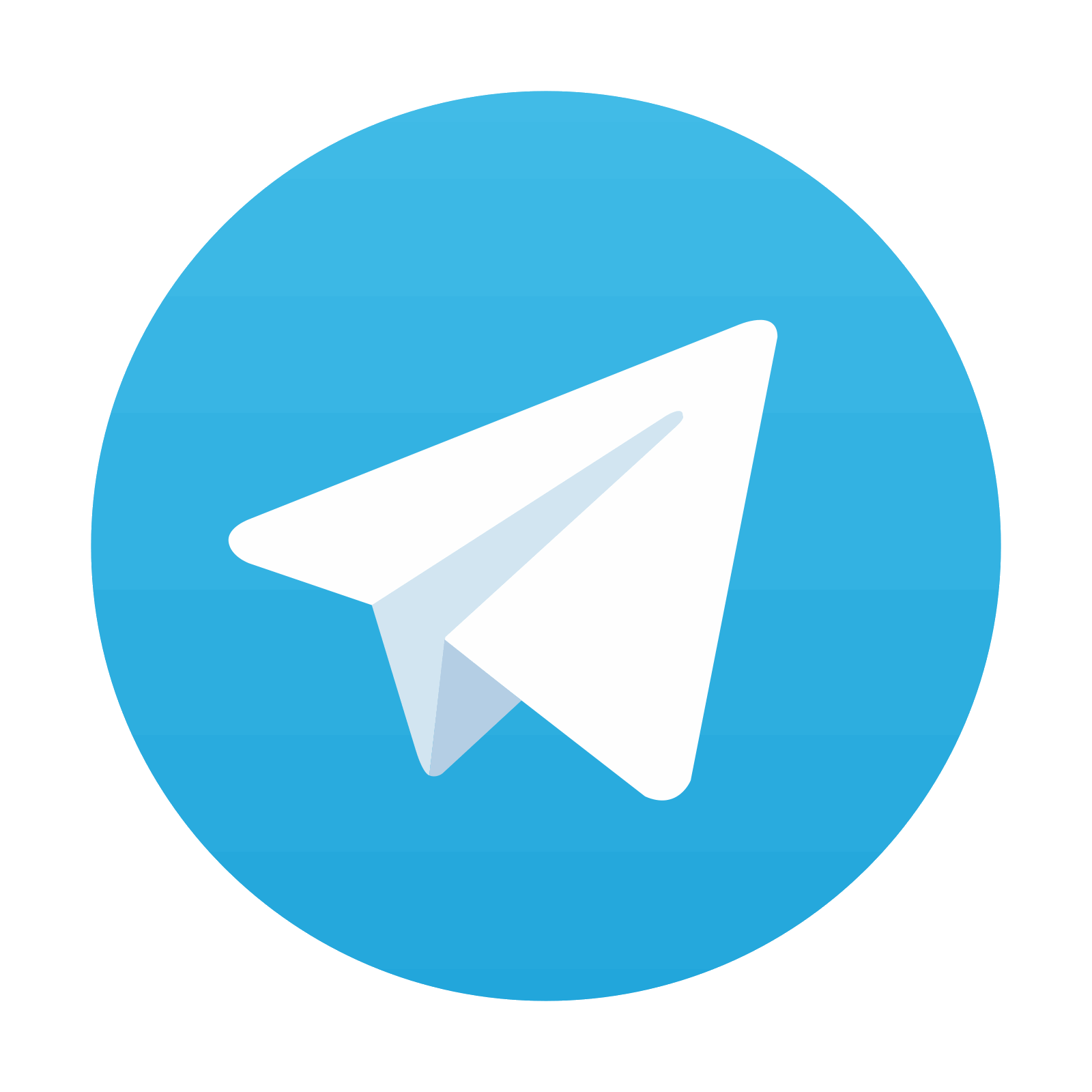
Stay updated, free articles. Join our Telegram channel
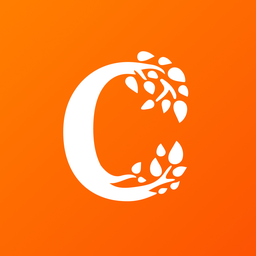
Full access? Get Clinical Tree
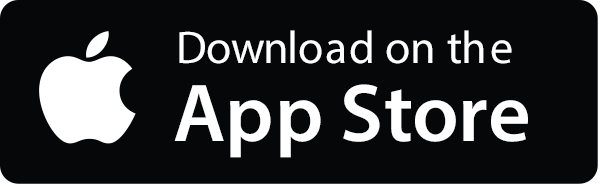
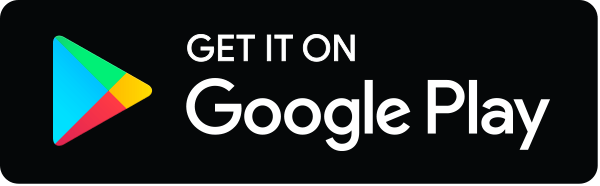