OBJECTIVES
After studying this chapter, youshould be able to:
Describe the main morphologic features of synapses.
Distinguish between chemical and electrical transmissions at synapses.
Describe fast and slow excitatory and inhibitory postsynaptic potentials, outline the ionic fluxes that underlie them, and explain how the potentials interact to generate action potentials.
Define and give examples of direct inhibition, indirect inhibition, presynaptic inhibition, and postsynaptic inhibition.
Describe the neuromuscular junction, and explain how action potentials in the motor neuron at the junction lead to contraction of the skeletal muscle.
Define denervation hypersensitivity.
INTRODUCTION
The “all-or-none” type of conduction seen in axons and skeletal muscle has been discussed in Chapters 4 and 5. Impulses are transmitted from one nerve cell to another cell at synapses. These are the junctions where the axon or some other portion of one cell (the presynaptic cell) terminates on the dendrites, soma, or axon of another neuron (Figure 6–1) or, in some cases, a muscle or gland cell (the postsynaptic cell). Cell-to-cell communication occurs across either a chemical or an electrical synapse. At chemical synapses, a synaptic cleft separates the terminal of the presynaptic cell from the postsynaptic cell. An impulse in the presynaptic axon causes secretion of a chemical that diffuses across the synaptic cleft and binds to receptors on the surface of the postsynaptic cell. This triggers events that open or close channels in the membrane of the postsynaptic cell. In electrical synapses, the membranes of the presynaptic and postsynaptic neurons come close together, and gap junctions are formed between the cells (see Chapter 2). Like the intercellular junctions in other tissues, these junctions form low-resistance bridges through which ions can pass with relative ease. There are also a few conjoint synapses in which transmission is both electrical and chemical.
FIGURE 6–1
Synapses on a typical motor neuron. The neuron has dendrites (1), an axon (2), and a prominent nucleus (3). Note that rough endoplasmic reticulum extends into the dendrites but not into the axon. Many different axons converge on the neuron, and their terminal boutons form axodendritic (4) and axosomatic (5) synapses. (6) Myelin sheath is a protein-lipid complex that wraps around the axon. (Reproduced with permission from Krstic RV: Ultrastructure of the Mammalian Cell. Springer, 1979.)
Regardless of the type of synapse, transmission is not a simple transmission of an action potential from the presynaptic to the postsynaptic cell. The effects of discharge at individual synaptic endings can be excitatory or inhibitory, and when the postsynaptic cell is a neuron, the summation of all the excitatory and inhibitory effects determines whether an action potential is generated. Thus, synaptic transmission is a complex process that permits the grading and adjustment of neural activity necessary for normal function. Because most synaptic transmission is chemical, consideration in this chapter is limited to chemical transmission unless otherwise specified.
Transmission from nerve to muscle resembles chemical synaptic transmission from one neuron to another. The neuromuscular junction, the specialized area where a motor nerve terminates on a skeletal muscle fiber, is the site of a stereotyped transmission process. The contacts between autonomic neurons and smooth and cardiac muscle are less specialized, and transmission in these locations is a more diffuse process. These forms of transmission are also considered in this chapter.
SYNAPTIC TRANSMISSION: FUNCTIONAL ANATOMY
The anatomic structure of synapses varies considerably in the different parts of the mammalian nervous system. The ends of the presynaptic fibers are generally enlarged to form terminal boutons or synaptic knobs (Figure 6–2). In the cerebral and cerebellar cortex, endings are commonly located on dendrites and frequently on dendritic spines, which are small knobs projecting from dendrites (Figure 6–3). In some instances, the terminal branches of the axon of the presynaptic neuron form a basket or net around the soma of the postsynaptic cell (eg, basket cells of the cerebellum). In other locations, they intertwine with the dendrites of the postsynaptic cell (eg, climbing fibers of the cerebellum) or end on the dendrites directly (eg, apical dendrites of cortical pyramidal cells). Some end on axons of postsynaptic neurons (axoaxonal endings). On average, each neuron divides to form over 2000 synaptic endings, and because the human central nervous system (CNS) has 1011 neurons, it follows that there are about 2 × 1014 synapses. Obviously, therefore, communication between neurons is extremely complex. Synapses are dynamic structures, increasing and decreasing in complexity and number with use and experience.
It has been calculated that in the cerebral cortex, 98% of the synapses are on dendrites and only 2% are on cell bodies. In the spinal cord, the proportion of endings on dendrites is less; there are about 8000 endings on the dendrites of a typical spinal neuron and about 2000 on the cell body, making the soma appear encrusted with endings.
Each presynaptic terminal of a chemical synapse is separated from the postsynaptic structure by a synaptic cleft that is 20–40 nm wide. Across the synaptic cleft are many neurotransmitter receptors in the postsynaptic membrane, and usually a postsynaptic thickening called the postsynaptic density (Figures 6–2 and 6–3). The postsynaptic density is an ordered complex of specific receptors, binding proteins, and enzymes induced by postsynaptic effects.
FIGURE 6–3
Axodendritic, axoaxonal, and axosomatic synapses. Many presynaptic neurons terminate on dendritic spines, as shown at the top, but some also end directly on the shafts of dendrites. Note the presence of clear and granulated synaptic vesicles in endings and clustering of clear vesicles at active zones.
Inside the presynaptic terminal are many mitochondria, as well as many membrane-enclosed vesicles, which contain neurotransmitters. There are three kinds of synaptic vesicles: small, clear synaptic vesicles that contain acetylcholine, glycine, GABA, or glutamate; small vesicles with a dense core that contain catecholamines; and large vesicles with a dense core that contain neuropeptides. The vesicles and the proteins contained in their walls are synthesized in the neuronal cell body and transported along the axon to the endings by fast axoplasmic transport. The neuropeptides in the large dense-core vesicles must also be produced by the protein-synthesizing machinery in the cell body. However, the small clear vesicles and the small dense-core vesicles recycle in the nerve ending. These vesicles fuse with the cell membrane and release transmitters through exocytosis and are then recovered by endocytosis to be refilled locally. In some instances, they enter endosomes and are budded off the endosome and refilled, starting the cycle over again. The steps involved are shown in Figure 6–4. More commonly, however, the synaptic vesicle discharges its contents through a small hole in the cell membrane, then the opening reseals rapidly and the main vesicle stays inside the cell (kiss-and-run discharge). In this way, the full endocytotic process is short-circuited.
FIGURE 6–4
Small synaptic vesicle cycle in presynaptic nerve terminals. Vesicles bud off the early endosome and then are filled with a neurotransmitter (NT). The vesicles then translocate toward release sites on the plasma membrane where they dock, and then become primed. Following arrival of an action potential at the nerve ending, Ca2+ influx triggers fusion and exocytosis of the vesicular contents into the synaptic cleft. The vesicle wall then is coated with clathrin, which likely facilitates endocytosis. Once back inside the cytoplasm, the vesicle becomes uncoated and fuses with the early endosome to start the cycle again.
The large dense-core vesicles are located throughout the presynaptic terminals that contain them and release their neuropeptide contents by exocytosis from all parts of the terminal. On the other hand, the small vesicles are located near the synaptic cleft and fuse to the membrane, discharging their contents very rapidly into the cleft at areas of membrane thickening called active zones (Figure 6–3). The active zones contain many proteins and rows of Ca2+ channels.
The Ca2+ that triggers exocytosis of transmitters enters the presynaptic neurons, and transmitter release starts within 200 μs. Therefore, it is not surprising that the voltage-gated Ca2+ channels are very close to the release sites at the active zones. In addition, the transmitter must be released close to the postsynaptic receptors to be effective on the postsynaptic neuron. This orderly organization of the synapse depends in part on neurexins, the proteins bound to the membrane of the presynaptic neuron that bind neurexin receptors in the membrane of the postsynaptic neuron. In many vertebrates, neurexins are produced by a single gene that codes for the α isoform. However, in mice and humans they are encoded by three genes, and both α and β isoforms are produced. Each of the genes has two regulatory regions and extensive alternative splicing of their mRNAs. In this way, over 1000 different neurexins are produced. This raises the possibility that the neurexins not only hold synapses together, but also provide a mechanism for the production of synaptic specificity.
As noted in Chapter 2, vesicle budding, fusion, and discharge of contents with subsequent retrieval of vesicle membrane are fundamental processes occurring in most, if not all, cells. Thus, neurotransmitter secretion at synapses and the accompanying membrane retrieval are specialized forms of the general processes of exocytosis and endocytosis. The fusion of synaptic vesicles with the cell membrane involves the action of several proteins, including the N-ethylmaleimide–sensitive fusion protein (NSF), SNAPs (soluble NSF attachment proteins), and SNAREs (SNAP receptors; Figure 6–5). Synaptobrevin in the vesicle membrane locks with syntaxin and SNAPs in the cell membrane; GTPases regulate a multiprotein complex that includes Rab and Sec1/Munc18-like proteins as part of the fusion process. The one-way gate at the synapses is necessary for orderly neural function.
FIGURE 6–5
Main proteins that interact to produce synaptic vesicle docking and fusion in nerve endings. Several proteins are involved in synaptic vesicle docking and fusion, including the N-ethylmaleimide–sensitive fusion protein (NSF), SNAPs (soluble NSF attachment proteins), and SNAREs (SNAP receptors). Synaptobrevin in the vesicle membrane links with syntaxin and SNAPs in the cell membrane; GTPases regulate a multiprotein complex that includes Rab and Sec1/Munc18-like proteins.
Several deadly toxins that block neurotransmitter release are zinc endopeptidases that cleave and hence inactivate proteins in the fusion-exocytosis complex. Clinical Box 6–1 describes how neurotoxins from bacteria called Clostridium tetani and Clostridium botulinum can disrupt neurotransmitter release in either the CNS or at the neuromuscular junction.
ELECTRICAL EVENTS IN POSTSYNAPTIC NEURONS
Penetration of an α-motor neuron is a good example of a technique used to study postsynaptic electrical activity. It is achieved by advancing a microelectrode through the ventral portion of the spinal cord. Puncture of a cell membrane is signaled by the appearance of a steady 70 mV potential difference between the microelectrode and an electrode outside the cell. The cell can be identified as a spinal motor neuron by stimulating the appropriate ventral root and observing the electrical activity of the cell. Such stimulation initiates an antidromic impulse (see Chapter 4) that is conducted to the soma and stops at that point. Therefore, the presence of an action potential in the cell after antidromic stimulation indicates that the cell that has been penetrated is an α-motor neuron. Stimulation of a dorsal root afferent (sensory neuron) can be used to study both excitatory and inhibitory events in α-motor neurons (Figure 6–6).
FIGURE 6–6
Excitatory and inhibitory synaptic connections mediating the stretch reflex provide an example of typical circuits within the CNS. A) The stretch receptor sensory neuron of the quadriceps muscle makes an excitatory connection with the extensor motor neuron of the same muscle and an inhibitory interneuron projecting to flexor motor neurons supplying the antagonistic hamstring muscle. B) Experimental setup to study excitation and inhibition of the extensor motor neuron. Top panel shows two approaches to elicit an excitatory (depolarizing) postsynaptic potential or EPSP in the extensor motor neuron–electrical stimulation of the whole Ia afferent nerve using extracellular electrodes and intracellular current passing through an electrode inserted into the cell body of a sensory neuron. Bottom panel shows that current passing through an inhibitory interneuron elicits an inhibitory (hyperpolarizing) postsynaptic potential or IPSP in the flexor motor neuron. (Reproduced with permission from Kandel ER, Schwartz JH, Jessell TM [editors]: Principles of Neural Science, 4th ed. New York, NY: McGraw-Hill; 2000.)
Once an impulse reaches the presynaptic terminals, a response can be obtained in the postsynaptic neuron after a synaptic delay. The delay is due to the time it takes for the synaptic mediator to be released and to act on the receptors on the membrane of the postsynaptic cell. Because of it, conduction along a chain of neurons is slower if there are many synapses compared to if there are only a few synapses. Because the minimum time for transmission across one synapse is 0.5 ms, it is also possible to determine whether a given reflex pathway is monosynaptic or polysynaptic (contains more than one synapse) by measuring the synaptic delay.
A single stimulus applied to the sensory nerves characteristically does not lead to the formation of a propagated action potential in the postsynaptic neuron. Instead, the stimulation produces either a transient partial depolarization or a transient hyperpolarization. The initial depolarizing response produced by a single stimulus to the proper input begins about 0.5 ms after the afferent impulse enters the spinal cord. It reaches its peak 11.5 ms later and then declines exponentially. During this potential, the excitability of the neuron to other stimuli is increased, and consequently the potential is called an excitatory postsynaptic potential (EPSP) (Figure 6–6).
The EPSP is produced by depolarization of the postsynaptic cell membrane immediately under the presynaptic ending. The excitatory transmitter opens Na+ or Ca2+ channels in the postsynaptic membrane, producing an inward current. The area of current flow thus created is so small that it does not drain off enough positive charge to depolarize the whole membrane. Instead, an EPSP is inscribed. The EPSP due to activity in one synaptic knob is small, but the depolarizations produced by each of the active knobs summate.
CLINICAL BOX 6–1 Botulinum and Tetanus Toxins
Clostridia are gram-positive bacteria. Two varieties, Clostridium tetani and Clostridium botulinum, produce some of the most potent biologic toxins (tetanus toxin and botulinum toxin) known to affect humans. These neurotoxins act by preventing the release of neurotransmitters in the CNS and at the neuromuscular junction. Tetanus toxin binds irreversibly to the presynaptic membrane of the neuromuscular junction and uses retrograde axonal transport to travel to the cell body of the motor neuron in the spinal cord. From there it is picked up by the terminals of presynaptic inhibitory interneurons. The toxin attaches to gangliosides in these terminals and blocks the release of glycine and GABA. As a result, the activity of motor neurons is markedly increased. Clinically, tetanus toxin causes spastic paralysis; the characteristic symptom of “lockjaw” involves spasms of the masseter muscle. Botulism can result from ingestion of contaminated food, colonization of the gastrointestinal tract in an infant, or wound infection. Botulinum toxins are actually a family of seven neurotoxins, but it is mainly botulinum toxins A, B, and E that are toxic to humans. Botulinum toxins A and E cleave synaptosome-associated protein-25 (SNAP-25). This is a presynaptic membrane protein needed for fusion of synaptic vesicles containing acetylcholine to the terminal membrane, an important step in transmitter release. Botulinum toxin B cleaves synaptobrevin, a vesicle-associated membrane protein (VAMP). By blocking acetylcholine release at the neuromuscular junction, these toxins cause flaccid paralysis. Symptoms can include pto(drooping eyelid), diplopia (double vision), dysarthria (slurred speech), dysphonia (difficulty speaking), and dysphagia (difficulty swallowing).
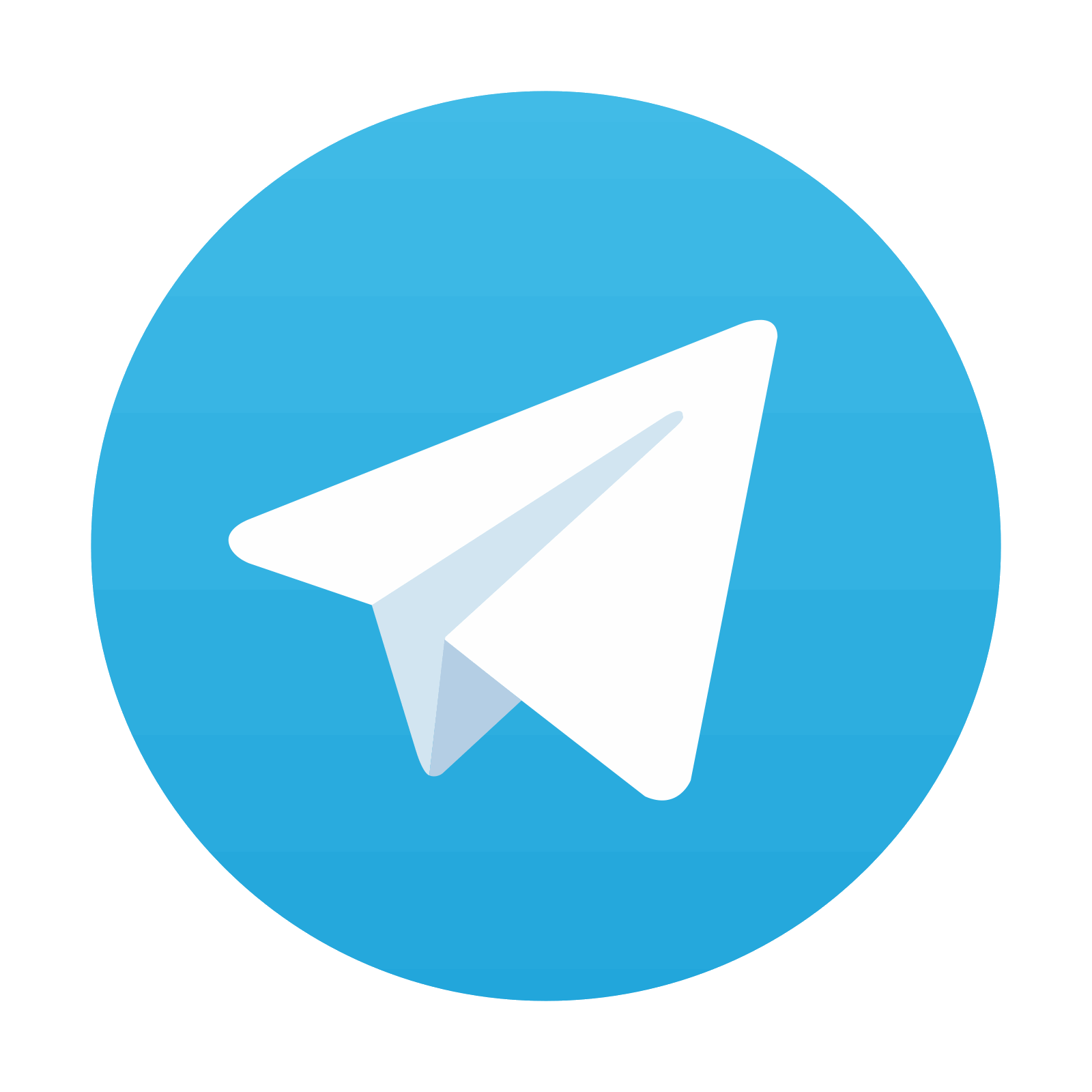
Stay updated, free articles. Join our Telegram channel
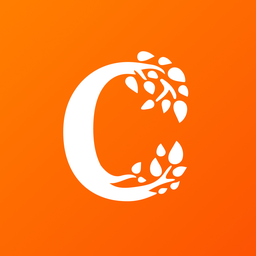
Full access? Get Clinical Tree
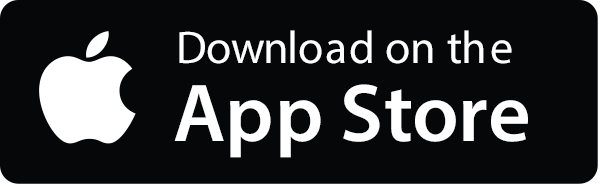
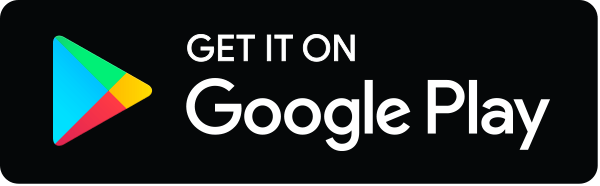
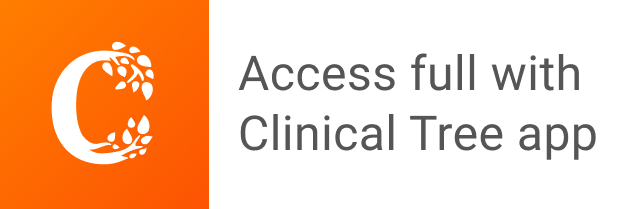