Fig. 6.1
Schematic representation of various layers in blood vessels
6.2 Important Signaling Molecules in Vasculogenesis and Angiogenesis
Understanding important signaling molecules at each level of development of arteries, veins and capillaries and their maturation could help us to engineer blood vessels that are a more faithful mimic of the natural system. Here, we review briefly the important molecules and pathways in vasculogenesis, angiogenesis, and maturation. Tissue engineering strategies may benefit from generating materials that can guide these biological events in the formation of vascular networks. How scientists and researchers use this information to engineer the blood vessels is discussed in Sect. 6.4.
6.2.1 Formation of Immature Vasculature by Vasculogenesis and Angiogenesis
During embryonic development, angioblasts migrate to various regions of the developing embryo and differentiate into ECs in response to local cues such as growth factors and ECM components. The ECs then form a vascular plexus, which is a network built by connections (anastomoses) between blood vessels. This process is called vasculogenesis and it is not limited to the embryonic period, as a similar process can also occur in adults through the recruitment and participation of bone marrow-derived endothelial progenitor cells [3, 7, 8].
Angiogenesis is another mechanism of blood vessel formation that occurs through the sprouting of existing blood vessels. Angiogenesis is a sequential, multistep process that begins with activation of a quiescent endothelium by proangiogenic factors such as vascular endothelial growth factor (VEGF), fibroblast growth factor (FGF), and angiopoietin-2 (Ang2) that are often produced by hypoxic or tumorigenic tissues [3, 8]. Hypoxia up-regulates expression of a number of genes involved in vessel formation, patterning, and maturation, including nitric oxide synthase, VEGF, and Ang2. Nitric oxide, which is a product of nitric oxide synthase, dilates vessels and make them more responsive to VEGF because they becomes more leaky. Ang2 also facilitates sprout formation in the presence of VEGF. The sprouts anastomose to form vascular loops and networks [3].
Angiogenesis is also dependent on degradation of the BM, a thin layer of ECM between the epithelial cell layer and the endothelial cell lining of blood vessels. Degradation of BM is due to up-regulation of matrix metalloproteinases (MMPs) such as MMP2, MMP3, and MMP9, and suppression of protease inhibitors such as tissue inhibitor of metalloproteinase-2 (TIMP2). BM degradation is followed by migration of an endothelial tip cell from the leading edge of a vascular sprout; this leading edge defines the direction of the newly growing sprout [2, 6, 7].
6.2.2 Endothelial Cell Branching and Proliferation
High levels of proangiogenic factors (such as VEGFA and VEGFC) and of VEGF receptor 2 (VEGFR2) or VEGFR3 signaling select “tip cells” (TCs) for sprouting during angiogenesis. By contrast, Delta-like 4-notch signaling laterally inhibits TC fate in adjacent ECs. TC sprouting behavior is facilitated by the vascular endothelial cadherin-mediated loosening of EC–EC junctions, matrix metalloproteinase-mediated degradation of ECM and the detachment of pericytes. Guidance of TC sprouting is due to the gradients of proangiogenic growth factors and various environmental guidance cues, such as semaphorins and ephrins. During sprout elongation, TCs are trailed by endothelial “stalk cells” (SCs), which maintain connectivity with parental vessels and initiate partitioning-defective3 (PAR3)-mediated vascular lumen morphogenesis. Expression of VEGFR1 and activation of notch, Roundabout homologue 4 and WNT signaling in SCs repress TC behavior to maintain the hierarchical organization of sprouting ECs. However, TCs and SCs may also shuffle and exchange positions during angiogenic sprouting. Upon contact with other vessels, TC behavior is repressed and vessels fuse by the process of anastomosis, which is assisted by associated myeloid cells. The ECs adjacent to the tip cells begin to proliferate and elongate to form capillary sprouts, which then assemble to form a vessel lumen. After the activation and proliferation stages, a nascent blood vessel must mature to become functional [6–11].
6.2.3 Stabilization of Immature Vasculature
After the activation and proliferation stages, a nascent blood vessel must mature to become functional. The nascent vessels are stabilized by recruiting mural cells and by deposition of an ECM, in a process known as arteriogenesis. There are at least four molecular pathways that regulate this process, including the following:
1.
Platelet-derived growth factor PDGFB and PDGF receptor (PDGFR)-β.
2.
Sphingosine-1-phosphate-1 (S1P)-endothelial differentiation sphingolipid G-protein-coupled receptor-1 (EDG1).
3.
Ang1-Tie2.
4.
Transforming growth factor TGF-β1.
Recruitment of mural cells such as pericytes and smooth muscle cells (SMCs) to the developing immature vasculature by platelet-derived growth factor B (PDGFB) and transforming growth factor-β1 (TGF-β1) stabilize the vessel wall [2, 7, 12, 13]. The contact between ECs and mural cells is strengthened by bioactive lipid spingosine1 phosphate (S1P) through activating the guanine nucleotide-binding-coupled receptor, and S1P receptor 1(S1PR1 or EDG1) signaling [7, 14]. Tie receptors (Tie1 and Tie2) and their ligands Ang1 and Ang2 are also critical for vessel formation and stabilization. Main sources of Ang1 and Ang2 are the mural cells and ECs, respectively. Ang1 stabilizes nascent vessels by facilitating communication between ECs and mural cells. Ang2 acts as an antagonist of Ang1 in the absence of VEGF and destabilizes vessels in the presence of VEGF [15].
TGF-β1 is a multifunctional growth factor that promotes vessel maturation by stimulating ECM production and by inducing differentiation of mesenchymal cells to mural cells. It is expressed in a number of cell types, including ECs and mural cells, and depending on the context and concentration, could be pro- or anti-angiogenic. Recent in vitro studies indicate that the TGF-β1–ALK1 pathway is a positive regulator of endothelial cell migration and proliferation by inducing ECs and fibroblasts to express Id1, a protein required for proliferation and migration. On the other hand, the TGF-β1–ALK5 pathway is a positive regulator of vessel maturation by inducing the plasminogen activator inhibitor (PAI1) in ECs. PAI1 promotes vessel maturation by preventing degradation of the provisional matrix around the nascent vessel. Thus, the degree to which TGF-β signals through ALK1 versus ALK5 can determine the pro- or anti-angiogenic effect of TGF-β [2, 10]. Figure 6.2 shows important signaling molecules that are involved in vasculogenesis, maturation of blood vessels and angiogenesis.
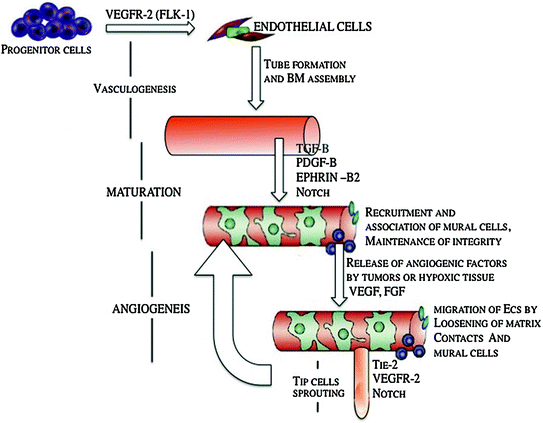
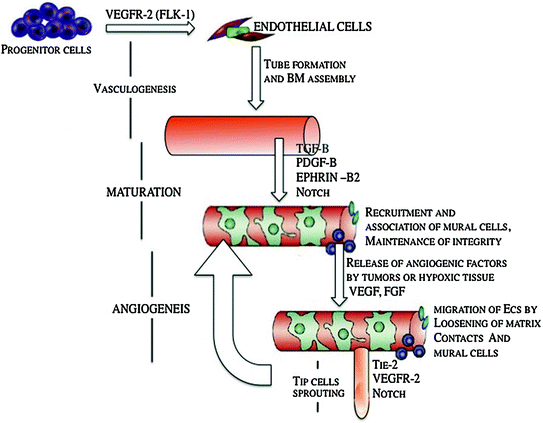
Fig. 6.2
Schematic of important signaling molecules that are involved in vasculogenesis, maturation of blood vessels, and angiogenesis, modified from Ref. [6]
6.2.3.1 Role of Basement Membrane in Stabilization of Immature Blood Vessels
As mentioned earlier, BM degradation happens during the complicated multistep angiogenesis process. Direct contact between the BM and the EC layer provides important signals that control the stability of EC layer and facilitate EC tube stabilization. Vascular basement membrane matrices are largely composed of structural components, including laminin (particularly laminin IV), collagen IV, and fibronectin. Other proteins provide bridging functions such as nidogens 1 and 2, and the heparan sulfate proteoglycan perlecan, which facilitate the co-assembly of BM components [16–18]. It has long been known that ECs have the capacity to synthesize most if not all of these proteins, so it was generally assumed that BM assembly occurred through ECs alone. However, in a variety of tissues, most notably the skin, it is clear that BM assembly requires more than keratinocytes and was strongly stimulated by the presence of fibroblasts in collagenous matrices underlying the keratinocyte layer. Thus, by analogy with these findings, it is likely that vascular basement membrane assembly may require heterotypic cell–cell contacts, which was originally suggested by Davis and Senger [19]. A recent study by Stratman et al. demonstrates that pericyte recruitment to EC-lined tubes in vitro and in vivo is necessary to stimulate vascular basement membrane matrix assembly, a key step in vascular maturation and stabilization [20].
Collectively, the matrix serves as a store for various growth factors and proenzymes involved in vessel development. The balance between proteases (such as MMP2, MMP3, MMP9, and urokinase plasminogen activator) and their inhibitors (such as tissue inhibitors of metalloproteinases and PAI1) controls BM and ECM degradation and could influence EC and mural cell migration [7, 20, 21]. These proteases also lead to the release of various proangiogenic growth factors, such as VEGF and basic fibroblast growth factor (bFGF), which are sequestered in the matrix. Protease activity can also generate anti-angiogenic molecules by cleaving plasma proteins (such as angiostatin from plasminogen), matrix molecules (such as tumstatin from collagen type IV), or the proteases themselves (such as PEX from MMP2). Thus, branching patterns of vessels are tightly regulated by spatial and temporal concentration profiles of growth factors and protein fragments that transport and bind to the matrix [2, 4].
6.3 Sources of Endothelial Cells and Their Progenitors
One potential source of ECs are embryonic stem cells, which are pluripotent and thus capable of differentiating into all cell types of the endoderm, ectoderm, and mesoderm. Although ESCs have the advantages of greater proliferative capacity and pluripotentiality when compared to other endothelial cell precursors, these properties also raise a concern. Specifically, the inadvertent administration of an undifferentiated (and thus pluripotent) ESC to a patient would risk teratoma formation. Accordingly, the clinical development of this cell therapy will require robust differentiation and purification protocols, supported by data showing the safety of these cells. The therapeutic use of these cells is further complicated because they are allogeneic and therapeutic engraftment may require immunosuppression, which carries additional risk. Finally, the clinical use of these cells may be influenced by the ethical debate surrounding the isolation of cells from human embryos. Accordingly, there is great interest in a new form of pluripotential cell that can obviate some of these concerns. Induced pluripotent stem cells (iPSCs) can be “reprogrammed” from adult somatic cells using a variety of methods, established primarily by Yamanaka and Thomson. iPSCs have the potential to generate patient-specific tissues for disease modeling and regenerative medicine applications. However, before iPSC technology can progress to the translational phase, several obstacles must be overcome. These include uncertainty regarding the ideal somatic cell type for reprogramming, the low kinetics and efficiency of reprogramming, and karyotype discrepancies between iPSCs and their somatic precursors. In this section, we describe different sources of ECs and common endothelial cell markers that have been investigated so far by researchers, from embryonic and iPSCs to adult cells.
6.3.1 Embryonic Stem Cell-Derived Endothelial Cells
From Embryoid Bodies (EB)
Mouse embryonic stem cells (mESCs) can differentiate into hemangioblasts after forming embryoid bodies (EBs). Hemangioblasts that form blood islands contain endothelial and haematopoietic progenitors [22]. Formation of a vessel-like network is the result of further differentiation of the EBs [23]. Moreover, it has been shown that endothelial cell markers differentiated from EBs are expressed in the same order that is expressed in endothelial differentiation during embryonic development [24].
During human EB differentiation, endothelial cell markers such as CD31, CD34, VE-cad ,and GATA-2 show increasing trends in their expression [25, 26]. CD31, CD34, and VE-cad reach a maximum at days 13–15 and GATA-2 around day 18. Other endothelial markers such as VCAM1, FLT-1, FLT-2; vasculogenic growth factors such as VEGF, Ang1, Ang2, and PDGF; and transcription factors such as GATA1 and GATA3 are up-regulated as well [27]. Like mouse ESCs, human ESCs (hESCs) can spontaneously differentiate and organize within EBs into three-dimensional vessel-like structures, in a pattern that resembles embryonic vascularization. The capillary area in the human EBs increases during subsequent maturation steps, starting from cell clusters that later sprout into capillary-like structures and eventually organize in a network-like arrangement. These isolated CD31+ cells from human EBs (days 13–15), express endothelial markers and can form vascular tubes in vitro and in vivo [28].
On feeder layer and ECM
Seeding ES cells on feeder cells or within an ECM can also induce differentiation into haematopoietic and endothelial lineages. For example, mouse endothelial progenitors (Flk-1+ cells) were isolated following the differentiation of ESCs on collagen. The isolated Flk-1+ cells in mouse systems are precursors for haematopoietic, endothelial, and SMCs and can also give rise to contracting cardiac cells, thus acting as cardiohemangioblasts [29, 30]. Culturing of human embryonic stem cells (hESC) on collagen IV resulted in two types of cell population that differ by size. The smaller cell population showed an upregulation of specific endothelial markers, such as CD31, CD34, Tei2, and GATA2. Cord-like organization of the cells (20 % ECs) was observed by re-plating the smaller population of cells on collagen IV with VEGF supplementation. Moreover, addition of PDGFB induced differentiation into SMCs [31]. Seeding hESCs on stromal feeder cells (bone marrow and yolk sac) could lead to differentiation into CD34+ cells (1–2 %). Interestingly, about 50 % of the CD34+ cells also express CD31. The CD34+ cells were isolated and differentiated into haematopoietic cells [32].
6.3.2 Adult-Derived Endothelial Cells
Human umbilical vein endothelial cells (HUVECs) show relatively higher proliferative potential among the isolated CD31+ ECs that originate from veins and arteries of different tissues [28]. Isolation of CD34+ and Flk-1+ cells from peripheral blood using magnetic beads is another source of adult ECs [16]. These isolated progenitor cells could differentiate into ECs and incorporate into neovascularization sites in mouse and rabbit hindlimb ischemic models [34]. CD34+ cells, mobilized from the bone marrow following treatment with granulocyte macrophage colony stimulating factor, improved ventricular function and neoangiogenesis in ischemic nude rat myocardium [35]. CD133+ cells purified from bone marrow were also shown to enhance human myocardial perfusion and global function [36]. Another important source of adult ECs is the umbilical cord blood, which contains more CD133+ and CD34+ cells than adult peripheral blood and has higher proliferation capacity [37].
6.3.3 Induced Pluripotent Stem Cell-Derived Endothelial Cells
Yamanaka et al. have shown systematic differentiation of cardiovascular cells from mouse iPSCs. Induced pluripotent stem (iPS) cells were generated from mouse skin fibroblasts by introducing four transcription factors (Oct3/4, Sox2, Klf4, c-myc), and then, the same approach was applied on ES cells to induce cardiovascular differentiation. They showed that Flk1+ cells could differentiate into artery, vein, and mural cells [38]. Rufaihah et al. have differentiated human iPSCs (hiPSCs) into endothelial cells (hiPSC-ECs) to assess their ability to improve perfusion in a murine model of peripheral arterial disease [39, 40]. In brief, endothelial differentiation was initiated by culturing hiPSCs for 14 days in differentiation media supplemented with bone morphogenetic protein 4 and VEGF. They purified the heterogenous mixture of cells by FACS using an antibody directed against CD31. The purified hiPSC-ECs generated capillary-like structures when grown in Matrigel and incorporated acetylated-LDL cholesterol. These cells expressed endothelial markers such as FLK-1 (KDR), CD31, CD144, and eNOS. When exposed to hypoxia, the hiPSC–ECs produced angiogenic cytokines and growth factors. Subsequently, they transduced the cells with a double-fusion construct comprising firefly luciferase for BLI and green fluorescence protein for histochemistry. The hiPSC–ECs were administered on days 0 and 7 after femoral artery ligation into the ischemic hindlimb of immunodeficient mice. Over a two-week period, BLI revealed reduction of cells, but some hiPSC–ECs survived in the ischemic limb for at least 2 weeks. At that time, and for up to 4 weeks, perfusion was improved by over 30 % by comparison to the vehicle-treated group, as assessed by laser Doppler imaging. This effect was associated with a 60 % increase in the total number of capillaries in the ischemic limb of mice receiving hiPSC–EC injections by comparison to the vehicle-treated group. This preclinical work provided proof-of-concept for the use of hiPSC–EC in peripheral arterial disease [41].
Figure 6.3 shows development of therapeutic cells from human-induced pluripotent cells. Readily accessible somatic cells (e.g., skin fibroblasts) are harvested from a patient and expanded in culture. Cells are exposed to reprogramming transcriptional factors, in the form of cell permeant peptides and modified mRNA. The resulting iPSC colonies are differentiated into vascular progenitor cells, that are administered directly to patients with vascular disease, or which are incorporated into matrices as a biological conduit such as cylindrical bioengineered matrix or decellularized cadaveric vessels for surgical implantation. These bioengineered conduits would serve to replace autologous saphenous vein in patients that have insufficient or diseased veins [41].
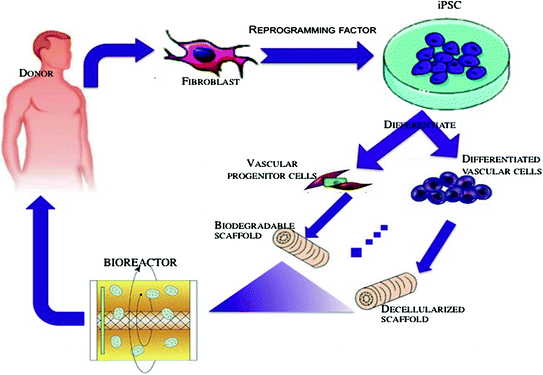
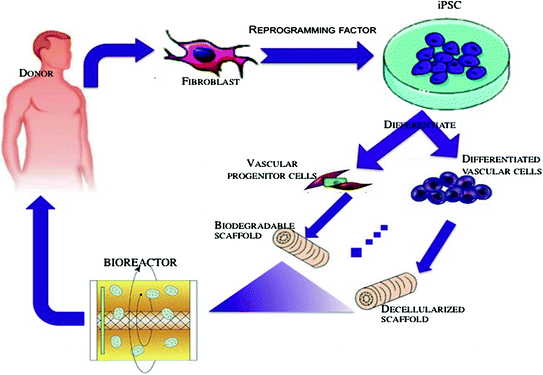
Fig. 6.3
Development of therapeutic cells from human-induced pluripotent cells
6.4 Engineering Blood Vessels from Stem Cell-Derived Endothelial Cells: Recent Advances and Applications
In the last three sections, we introduced the structure of blood vessels, important cell signaling molecules in vasculogenesis/angiogenesis, and different cell sources for ECs. Now, we will describe the tissue engineering approaches are available for making macrovessels and microvessels.
6.4.1 Approaches to Macrovessel Tissue Engineering (e.g., Heart Valve)
The approaches to engineer macrovessels can be divided into several distinct categories, although there may be some overlapping features. Successful application of these approaches, either individually or in combination, is expected to enhance therapeutic opportunities by building functional tissue and organ systems for regenerative medicine.
1.
Formation of a tissue in vitro by seeding cells on a biodegradable scaffold and maturing a tissue (to be implanted in vivo) in a bioreactor.
2.
Cell-seeded natural biodegradable scaffolds.
3.
Guided tissue regeneration via implanted degradable tissue that is remodeled by endogenous cells.
4.
Implantation of decellularized valvular material.
5.
Other approaches such as microfabrication techniques and scaffold-free approaches.
In all of the mentioned approaches, choosing the right cell among the spectrum of stem cells to differentiated cells and also the right scaffold is of utmost importance [42]. The scaffold should provide the initial requisite mechanical strength to withstand in vivo hemodynamic forces until vascular SMCs and fibroblasts reinforce the ECM of the vessel wall. Hence, the choice of scaffold is crucial for providing guidance cues to the cells to behave in the required manner to produce tissues and organs of the desired shape and size. Several types of scaffolds have been used for the reconstruction of blood vessels. They can be broadly classified as biological scaffolds, decellularized matrices, and polymeric biodegradable scaffolds. A review written by Pankajakhshan et al. focuses on the different types of scaffolds that have been designed, developed, and tested for tissue engineering of blood vessels [43].
6.4.1.1 Bioreactor Approach
The bioreactor approach should provide a desirable physiological, metabolic, and mechanical environment for blood vessel formation in vitro. To develop well-developed and effective construct for implantation and remodeling one approach would be to optimize the cellular component, the scaffolds, and the in vitro process conditions. Novel scaffolds are also under investigation. Multiple studies have emphasized that dynamic conditioning using bioreactors that provide a flow regime that mimics that of the intended application enhances construct tissue properties [44, 45]. Oxygen tension might be a key parameter for the achievement of sufficient tissue quality and mechanical integrity in tissue-engineered heart valves [46, 47]. A mesenchymal stem cell-seeded, valve-shaped construct has been assembled from layered collagenous scaffolds. Autologous fibrin-based engineered heart valves showed favorable results both in vitro and in vivo [48, 49]. Culturing vascular cells on polymer scaffolds and subjecting the scaffold to pulsating flow is another approach that is studied by Niklason et al. [50]. In fact, they seeded bovine aortic SMCs into hollow tubular polyglycolic acid (PGA) scaffolds and then injected bovine aortic ECs into the lumen. Compared with native arteries, the engineered arteries demonstrate similarities in wall thickness and collagen content after eight weeks of culture in a bioreactor. A key issue moving forward will be the real-time noninvasive and nondestructive assessment of mechanical properties of engineered heart valves both in vitro and utilization of such techniques in vivo to ensure quality.
6.4.1.2 Decellularized Vascular Material
Removal of all viable cells while preserving the ECM integrity is the goal in producing decellularized xenogeneic tissue. This method provides valuable material for heart valve tissue engineering. Decellularized materials have the advantage of preserving ECM components that may support cell adhesion and molecular sequestering, as well as desirable mechanical properties. However, ECM disruption by the decellularization process and immunogenicity are concerns inherent in the use of decellularized ECMs. For instance, decellularization resulted in substantial microscopic disruption of the ECM, which may negatively impact the durability of heart valve leaflets [51]. Moreover, evaluation of the relative immune responses of different valve components of decellularized porcine aortic valve compared with native and glutaraldehyde fixed valves showed that collagen I elicited a strong response but elastin induced a minimal response [52]. Recellularization of these valves has been reported in an aortic valve replacement model in juvenile pigs [53]. Another study investigated the function, histological changes, potential of in vivo re-endothelialization of decellularized aortic valve allografts in orthotopic position in sheep. The valves exhibited trivial regurgitation and normal morphology with no signs of graft dilatation, degeneration, or rejection [54]. Toxicity that is introduced to scaffold by the chemicals used in the decellularization process is another concern in decellularized natural ECMs. A clinical study investigated the safety and effectiveness of the Ross procedure (pulmonary to aortic valve autograft) using a decellularized, fibronectin-coated pulmonary valve allograft or xenograft seeded with autologous vascular ECs from a forearm or saphenous vein to reconstruct the right ventricular outflow tract. These valves showed excellent hemodynamic performance during midterm follow-up [55]. In contrast, recurrent right-sided heart failure after the Ross procedure was reported in a study done by Hiemann et al. [56].
6.4.1.3 Cell-Seeded Natural Biodegradable Scaffold
The cell-seeded natural biodegradable scaffold approach involves isolating and growing ECs on polymer scaffolds in vitro, followed by in vivo implantation. This method has been tested in an ovine model, where expanded pulmonary arterial cells were grown on polyglactin/poly (lactic-co-glycolic acid) tubular scaffolds for one week before transplantation into pulmonary arteries of lamb. Over a period of 24 weeks, the vascular grafts showed growth and development of endothelial lining and the production of ECM components, such as collagen and elastin fibers [57].
6.4.1.4 Microfabrication Technique
Another approach for the in vitro induction of endothelial networks in engineered tissue constructs is to prefabricate scaffolds to include channels that later could be lined with ECs. Microfabrication techniques are currently under way to engineer such network structures that will mimic the capillary network, expanding from a main vessel (like arteries), and merging back to a single vessel (like veins). In such systems, endothelial cells are seeded into the channel network and their attachment and behavior under flow are analyzed. Inkjet printing can be used to pattern cells into tubular structures. This technique, as well as other cell-printing techniques such as laser-guided direct writing, could be used in the future for the assembly of complex vascularized tissues. In one such approach, Tien and coworkers developed a method to prefabricate hollow channels within collagen gels. ECs were then seeded along the interior of the channel such that they formed a vessel-like structure that permitted flow of solution through the tube lumen. The authors demonstrated endothelial barrier function and appropriate barrier breakdown upon exposure to inflammatory cytokines [58].
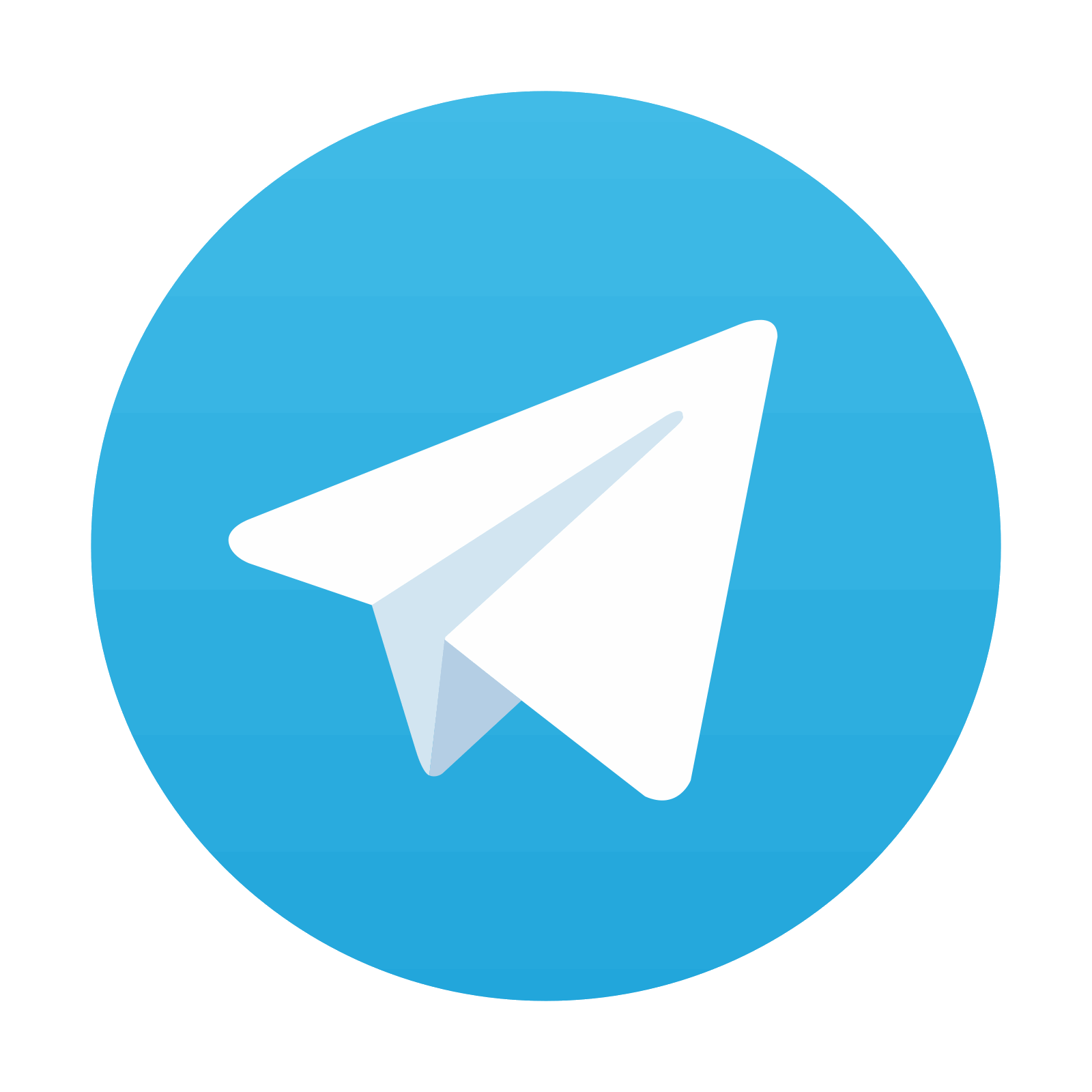
Stay updated, free articles. Join our Telegram channel
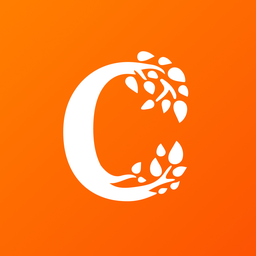
Full access? Get Clinical Tree
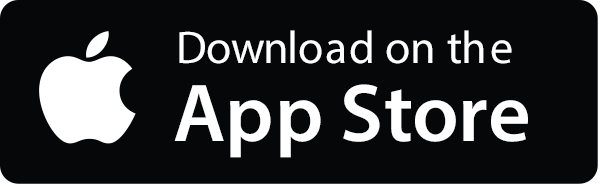
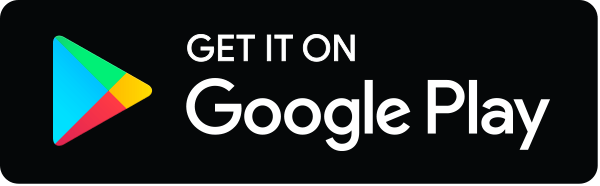