Structure and Function of the Nervous System
Jacquelyn L. Banasik
Key Questions
• How is the CNS protected and supported?
• What neurologic functions have been mapped to particular locations in the brain?
• How is the somatotopic organization of sensory receptors and muscles maintained in the CNS?
• How is voluntary muscle activity initiated and executed?
http://evolve.elsevier.com/Copstead/
The nervous system is a complex network of neurons and supportive cells that enables rapid communication between sensory receptors, central processing neurons, and functional responses. Much has been discovered about the mechanisms of sensory input and motor output, but the physiologic bases of thought, consciousness, emotion, and learning remain elusive. The idea that the mind is within the biological realm has been generally accepted, and the effects of mind-altering drugs on emotions, appetite, sleep, thought, and sensory perception have long been recognized. Research continues to reveal the great complexity of neurologic function. A bewildering array of neurotransmitter signaling molecules and an even greater number of neurotransmitter receptors have been identified. Recently, the long-held notion that neurons cannot regenerate in the mature brain has been disproved, and neuronal stem cells have been identified in certain areas. Each discovery brings us closer to understanding neural physiologic processes and gives hope for finding effective therapies for the devastating diseases that affect them. This chapter provides an overview of neural structure and function and is the basis for understanding the neurologic disorders in the chapters that follow.
Structural Organization
The nervous system is traditionally divided into three principal anatomic units: the central nervous system (CNS), the peripheral nervous system (PNS), and the autonomic nervous system (ANS). These systems are not anatomically or functionally distinct, and they work together as an integrated whole. Therefore, when function, rather than anatomy, is the topic of concern, the nervous system is more conveniently divided into the sensory, motor, and higher brain functions. This chapter begins with a review of the major anatomic features of the nervous system, then addresses neurologic function at the cellular and synaptic level, and concludes with a summary of sensory, motor, and cognitive functions.
Central Nervous System
The CNS includes the brain and spinal cord. Its primary functions are receiving and processing sensory information and creating appropriate responses to be relayed to muscles and glands. It is the site of emotion, memory, cognition, and learning. The CNS is bathed in cerebrospinal fluid (CSF) and shielded from the periphery by the blood-brain barrier. The CNS interacts with the neurons of the PNS through synapses in the spinal cord and cranial nerve ganglia. The cranial and spinal nerves constitute the PNS.
Support and Protection of the Central Nervous System
Nervous tissue has the consistency of gelatin, so measures to support and protect its fragile structure are necessary. In addition, the CNS must be shielded from circulating substances that would interfere with neurotransmission. These protective functions are provided by the skull and vertebral column, meninges, CSF, and blood-brain barriers. The bony structures of the skull and vertebral column encase the brain and cord and protect them from external trauma, whereas the CSF and meninges provide buoyancy and shock-absorbing capacity.
The meninges are composed of three layers that serve to suspend and maintain the shape and position of the nervous tissue during head and body movements. The brain is suspended within layers of meninges that are fixed to the skull. In this manner, the brain turns with the movement of the skull. The CSF circulates within the subarachnoid space, giving buoyancy to the brain and making an average 1500-gram brain mass resistant to distortion, which could occur from gravity alone were it not for the buoyancy effect.1 The three meningeal layers are the dura mater, arachnoid, and pia mater (Figure 43-1).
The dura mater, the outermost meningeal layer, is a thick, tough, collagenous membrane. It is composed of two layers, one contiguous with the periosteum of the skull and the other, which is adherent to the first, covering the surface of the brain. The tough dura protects the soft tissue of the brain. Support and stability are also provided by dural septa that invaginate into the cranial cavity. The falx cerebri is a thin wall of dura that folds down the cortical midline, separating the two hemispheres (see Figure 43-1). The tentorium cerebelli is a septum that separates the cerebellum and brainstem from the rest of the cerebrum. The dural septa fix the brain in place by their tentlike structure and limit its movement within the skull. Venous sinuses that collect venous blood from cerebral veins are located between the two layers of the dura at the base of the septum.
Beneath and continuous with the dura is the arachnoid layer. The spaces between the dura and the skull and between the dura mater and the arachnoid are potential spaces. Only in the presence of pathologic processes, notably epidural and subdural hemorrhages, do these spaces become evident (see Chapter 44). Unlike the dura mater, the arachnoid is a thin, delicate membrane. It is semitransparent and weblike in appearance, hence its name. Strands of collagenous connective tissue called trabeculae extend from the arachnoid layer down to the pia mater, forming a subarachnoid space. The CSF flows in this space.
The pia mater, the third meningeal layer, is also very thin. However, unlike the other meningeal layers, the pia is attached to the brain and closely follows its contours over every sulcus and into every gyrus. Consequently, the subarachnoid space between the arachnoid and the pia mater is not evenly distributed. The arachnoid meshes with the pia via the trabeculae in such a subtle manner that it is often difficult to differentiate one from the other. Consequently, the two layers together are often referred to as the leptomeninges.
The meninges that cover and provide protection to the spinal cord are similar to those of the brain, with a few variations (Figure 43-2). The spinal dura has no periosteal layer, so it is a single rather than a double layer. It is continuous with the foramen magnum at the base of the skull and is separated from the spinal vertebral periosteum by an epidural space. Thus, in the spinal cord, the epidural space is a true space, unlike its counterpart in the cranium, which is only a potential space. Within this space lie fatty connective tissue and a vertebral venous plexus.
The spinal arachnoid, much like that covering the cerebrum, is closely adherent to the spinal dura. Between the arachnoid layer and the pial lining is the CSF-filled subarachnoid space. The spinal meninges end at approximately the second sacral vertebra. However, the spinal cord ends between the first and second lumbar vertebrae (L1 to L2). This results in a large subarachnoid cistern, called the lumbar cistern, which is a favored place to obtain CSF samples (see Figure 43-2). The spinal pia is much tougher and thicker than the cerebral pia. Projecting along the length of each side is the dentate ligament, which anchors the spinal cord to the arachnoid and through it to the dura. Another pial projection connects the tail of the spinal cord (the cauda equina) at level L1 to L2 to the caudal end of the spinal dural sheath, where it is tethered to the end of the vertebral column. This projection is called the filum terminale.
The majority of CSF is produced by the choroid plexus, located in the lateral and third ventricles of the brain, at a rate of approximately 500 ml/day.2 The composition of normal CSF is compared to plasma in Table 43-1. CSF is absorbed at about the same rate at which it is produced, so that only 150 to 175 ml is in circulation at any time. The large C-shaped lateral ventricles occupy the center of each hemisphere. They communicate with the third ventricle in the diencephalon by way of the intraventricular foramen. The third ventricle is linked to the fourth ventricle by way of the cerebral aqueduct, which lies between the pons and the medulla (Figure 43-3). The CSF flows from the fourth ventricle through the median or lateral aperture and into the subarachnoid space. It flows around the spinal cord and up over the cerebral hemispheres to the arachnoid villi, where it is absorbed into the venous system.
TABLE 43-1
COMPOSITION OF CEREBROSPINAL FLUID COMPARED TO PLASMA AND SELECTED CEREBROSPINAL FLUID ABNORMALITIES
SUBSTANCE | NORMAL CSF | ABNORMAL CSF | PLASMA |
Na+ (mEq/L) | 148 | — | 136-145 |
K+ (mEq/L) | 2.9 | — | 3.5-5.0 |
Cl− (mEq/L) | 120-130 | — | 100-106 |
Glucose (mg/dl) | 50-75 | ↓ Infection | 70-100 |
Protein (mg/dl) | 15-45 | ↑ Inflammation | 6800 |
pH | 7.3 | — | 7.4 |
Red blood cells (high-power field) | None | ↑ Trauma, subarachnoid hemorrhage | — |
White blood cells (high-power field) | <5 | ↑ Infection (e.g., meningitis) | — |
Pressure (mm H2O) | 70-180 | ↑ Mass lesions | — |
CSF is absorbed by the arachnoid villi, which are small tufts of the arachnoid that invaginate into the dural sinus. These tufts bring CSF into close approximation with venous blood. CSF flows into the venous system through one-way valves because of pressure gradient differences. Although the CSF flows readily into the venous sinus, flow in the opposite direction cannot occur2; that is, the fluid in the venous sinus cannot flow into the subarachnoid space. This mechanism is part of a system of barriers between the extracellular space in the nervous system and the rest of the body.
The rate of production of CSF is independent of blood pressure or intraventricular pressure.2 Thus, CSF will continue to be produced even when its path of circulation or absorption is blocked. If this occurs, the amount of CSF increases, as does the size of the ventricles. This pathologic process is called hydrocephalus. Although hydrocephalus is usually caused by blockage of CSF pathways, it can also be caused by overproduction and malabsorption of CSF (see Chapter 45).
Blood supply to the brain is provided by two pairs of arteries; the anterior circulation is supplied by the internal carotid arteries, and the posterior circulation is supplied by the vertebral arteries (Figure 43-4). The cerebral circulation is discussed in detail in Chapter 44 as it relates to stroke. The internal carotid arteries have three principal branches: the anterior and middle cerebral arteries and the posterior communicating arteries. The vertebral arteries enter the skull at the foramen magnum and join at the level of the pons to form the basilar arteries. The ring of vessels that unites the anterior and posterior circulation at the base of the brain is known as the circle of Willis (see Figure 43-4, B). The cerebral veins drain into large vascular channels called sinuses that are formed by folds in the dura. From the sinuses, venous blood returns to the heart by way of the jugular veins (see Figure 43-4, C).
The extracellular fluid that bathes the neurons is carefully shielded from elements in the CSF and blood by cellular barriers. Specialized tight junctions between the cells that line the CSF spaces and between the endothelial cells of brain capillaries prevent leakage of molecules through the spaces between the cells (Figure 43-5). Therefore, substances must move through the plasma membranes of these barrier cells to access the CNS. Lipid-soluble molecules move through more easily than water-soluble ones. Thus, the flow of ions, nutrients, drugs, proteins, and other charged or polar substances is highly restricted.2 The blood-brain barrier (BBB) is a crucial structure for protecting the brain, but it may also restrict access of beneficial molecules, such as antibiotics and cancer drugs, making treatment more difficult.
The integrity of the blood-brain barrier is maintained in part by CNS cells called astrocytes. These specialized glial cells have foot processes that contact the brain capillaries and are thought to help regulate transport across the capillary endothelium3 (see Figure 43-5). The blood-brain barrier is less effective in infancy and can also be compromised by ischemia and chemical injury in adults.
A similar barrier exists between the circulating CSF and the interstitial fluid of the CNS—the CSF-brain barrier. The ependymal cells that line the ventricles are tightly joined and regulate the movement of water-soluble elements between the CSF and neurons. In addition, these cells serve the important function of removing unwanted substances from the CNS and secreting them into the CSF for eventual removal by the venous system.
Some areas of the brain need to sample the contents of the blood or CSF more directly to make regulatory adjustments in respiratory, autonomic, or endocrine functions, and these areas therefore have more permeable barriers (leaky BBB). These areas include the hypothalamus, pituitary, and other circumventricular organs (around the ventricles).
The Brain
Various schemes have been used to subdivide the structures of the brain using embryologic, evolutionary, and anatomic frameworks (Table 43-2). In this section, an anatomic framework is used that includes the cerebrum, diencephalon, cerebellum, and brainstem (Figure 43-6).
TABLE 43-2
SUBDIVISIONS OF THE BRAIN USING EMBRYOLOGIC, EVOLUTIONARY, AND ANATOMIC FRAMEWORKS
Framework | |||
STRUCTURE | EMBRYOLOGIC | EVOLUTIONARY | ANATOMIC |
Cerebral hemisphere | Telencephalon | Forebrain (includes diencephalon) | Cerebrum |
Thalamus | Diencephalon | — | Diencephalon hypothalamus |
Midbrain | Mesencephalon | Midbrain | — |
Cerebellum | Metencephalon (includes pons) | Cerebellum | Cerebellum |
Medulla | Myelencephalon | Hindbrain | Brainstem (includes midbrain, pons) |
Cerebrum
The cerebrum is divided into left and right hemispheres by the longitudinal fissure and is the largest part of the brain. The cerebral cortex is the outermost layer of the cerebrum and is composed of gray matter arranged in six histologically distinct layers4 (Figure 43-7). Each layer makes connections with other parts of the brain. The cortex is characterized by its convoluted exterior having ridges (gyri), grooves (sulci), and deeper depressions (fissures). The sulci and fissures are used as landmarks to divide the cerebral cortex into lobes. The central sulcus separates the frontal and parietal lobes, the lateral sulcus separates the temporal lobe from the parietal and frontal lobes, and the parieto-occipital line defines the occipital lobe (Figure 43-8).
Some anatomic locations are particularly associated with certain brain functions. These functions have been characterized through lesion studies in which an area of brain is damaged and then the functional losses studied, and by mapping procedures during which the cerebral cortex is stimulated and responses are recorded. The functional areas of specialization of brain loci are listed in Table 43-3. A partial map of Brodmann areas is shown in Figure 43-9. Although the concept of functional anatomic areas is clinically useful, one should realize that even though an area may be critical for a particular function, it is not wholly responsible for that function, and many brain areas may be involved. A certain degree of reassignment of brain function from one area to another can occur, allowing the brain to adapt to loss of normal neural function (neural plasticity).
TABLE 43-3
FUNCTIONAL AREAS OF BRAIN SPECIALIZATION
AREA | SPECIALIZED FUNCTION |
Occipital lobe | Visual cortex and association areas |
Parietal lobe | Somatosensory cortex and association areas |
Temporal lobe | Hearing and equilibrium, emotion, and memory |
Frontal lobe | Motor cortex and association areas; prefrontal cortex involved in complex thought, ethical behavior, and morality |
Limbic structures | Emotions, short-term memory, olfaction |
Basal ganglia | Initiation and planning of learned motor activities |
Broca and Wernicke areas | Interpretation and expression of language |
Functional areas of the cortex that can be mapped to specific sensory receptors or muscles are called primary areas. Primary areas are surrounded by secondary areas that provide greater character to sensations and greater complexity to movements. In addition to primary and secondary cortical areas, there are large areas of association cortex that add interpretive and learned responses. Organization of the primary and secondary cortex is best characterized for the somatosensory cortex and motor cortex (which are discussed later in the Somatosensory Cortex and the Central Control of Motor Function sections, respectively).
Cortical areas involved in visual perception are located in the occipital lobe. Brodmann area 17 is the primary visual cortex, and areas 18 and 19 are the secondary visual cortex. Interpretive association areas for vision are found in the adjacent temporal and parietal lobes. The primary auditory cortex is located on the superior temporal lobe, whereas vestibular information projects to the inferior temporal lobe.
Language expression and interpretation have been mapped to areas in the temporal lobe, particularly the Wernicke area (Brodmann areas 39, 40, and 22). One hemisphere, usually the left, is dominant for language. Lesions in this area lead to difficulty recognizing written words (alexia) and spoken language (receptive aphasia). Another area closely associated with speech is the Broca area (Brodmann area 44) in the frontal lobe. Damage to this region interferes with the ability to use language (expressive aphasia). In most cases, receptive and expressive aphasia occur together (see Chapter 44).
The frontal lobe is usually credited with control over emotional responses, ethical behavior, and morality. It is also the site of initiative and motivation. Patients with lesions of the frontal lobe may fail to conform to societal behavioral norms.
Another small cortical lobe, the central lobe or insula, lies deep in the lateral cerebral fissure under the junction of the frontal, parietal, and temporal lobes. Little is known about its specific functions, although it is thought to regulate visceral and intestinal functions.
The limbic lobe and limbic system are the parts of the cerebrum most closely associated with memory and emotion. The limbic lobe is a ring of cortex on the medial surface of each hemisphere containing the cingulate gyrus, isthmus, and parahippocampal gyrus (Figure 43-10). Olfaction (the perception of smell) occurs within the limbic cortex. The limbic system is a group of structures that encircle the brainstem. In addition to the limbic lobe, the limbic system includes the amygdala, fornix, hippocampus, and portions of the thalamus (see Figure 43-10). Lesions of the limbic system, particularly the hippocampus, cause impairment of short-term memory.
The basal ganglia are large masses of gray matter that lie deep within the cerebral hemispheres. They are intimately involved in the initiation, coordination, and execution of movement.5,6 The basal ganglia include the caudate nucleus, putamen, globus pallidus, subthalamus, and substantia nigra (Figure 43-11). The caudate nucleus and putamen together are called the striatum. The five basal ganglia structures occur in pairs, with each cerebral hemisphere containing a set.
The basal ganglia are connected by complex neural circuits that incorporate sensory information about the current muscle conditions, cortical input about desired motor activities, and cerebellar signals about timing and coordination. Much of what is known about the function of basal ganglia has been learned from studying Parkinson disease. Parkinson disease is characterized by difficulty initiating voluntary movements (akinesia), stiff muscles (rigidity), and a tremor of the hands when idle (rest tremor). Improvement in symptoms occurs when the patient is given a precursor of dopamine (DA, levodopa), which can cross the blood-brain barrier. Studies of these patients revealed degeneration of DA-secreting neurons that project from the substantia nigra to the striatum. As these neurons slowly degenerate over many years, the amount of DA secreted decreases, downstream γ-aminobutyric acid (GABA) pathways become dysregulated, and the relative activity of acetylcholine-secreting neurons in the basal ganglia is increased.7 A number of drugs (those that block DA) are known to produce a similar clinical syndrome (see Chapter 45).
In addition to the gray matter of the cerebral cortex and the basal ganglia, the cerebrum contains thick layers of white matter that consist of myelinated axons. Some of these axons connect the two cerebral hemispheres (commissural fibers), some connect one area of cortex to another within the same hemisphere (association fibers), and others connect the cortex with lower brain centers, including the thalamus, basal ganglia, brainstem, and spinal cord (projection fibers). The corpus callosum and the anterior commissure connect the two hemispheres. The corpus callosum is a massive bundle of fibers crossing the brain just above the lateral ventricles and is the principal means of communication between the hemispheres.
In summary, the cerebrum is the largest brain structure, garnering about 70% of the neurons and supporting cells of the brain to accomplish its diverse and complex functions. Each of the 100 billion neurons in the brain may make hundreds of synaptic connections with other neurons, providing an incomprehensible number of potential interactions. Discovering the ways in which the substance of the cerebrum relates to the workings of the mind is one of the great remaining mysteries of science.
Diencephalon
The diencephalon lies deep in the brain, forming a connecting structure between the upper brainstem (midbrain) and the cerebral hemispheres. The principal structures of the diencephalon are the thalamus, hypothalamus, pineal gland, epithalamus, and ventral thalamus (Figure 43-12). The third ventricle also traverses the diencephalon.
The thalamus is the principal receiving site and relay center for impulses traveling to the cerebral cortex from the spinal cord, cerebellum, and basal ganglia. In addition to processing and relaying sensory information, the thalamus is integrally involved in executing motor activities. The thalamus also is involved in propagating the constant background electrical activity of the brain, which can be detected by electroencephalography. Connections between the brainstem reticular activating system and thalamus are necessary to maintain consciousness. Thalamic connections, including the limbic and association cortex, are integral to the expression of those qualities considered to be human: emotion, language, creativity, and complex thought.8
The hypothalamus is located just beneath the thalamus on the floor of the diencephalon. The inferior aspect of the hypothalamus extends downward to form the pituitary gland (hypophysis). The posterior pituitary gland is an extension of the neuronal tissue of the hypothalamus, whereas the anterior pituitary gland is derived from glandular tissue (Figure 43-13). Hormones secreted by the pituitary gland enter the systemic circulation and influence target cells at a distance. Neurons in the hypothalamus regulate the secretion of anterior pituitary hormones by releasing and inhibiting hormones (see Chapter 39 for a discussion of the endocrine system).
The hypothalamus is also an important regulatory center for the ANS and for basic functions, such as sleep, body temperature, appetite, and sex drive. Input from sensors of blood pressure, osmolarity, blood oxygen concentration, carbon dioxide level and pH, and temperature is received and integrated into appropriate regulatory responses. The hypothalamus is responsible for homeostasis of life-sustaining functions, including cardiovascular, respiratory, metabolic, fluid and electrolyte, and stress responses.
The epithalamus contains the pineal gland, thought to be important in regulating circadian rhythms in response to light-dark cycles. The ventral thalamus contains the basal ganglia structure called the subthalamic nucleus.
Cerebellum
The cerebellum is located in the posterior fossa behind the pons. It is separated from the cerebrum by the tentorium cerebelli. The main roles of the cerebellum are to coordinate and smooth movements and to maintain posture and balance. The cerebellum compares the desired motor program with the moment-to-moment execution of the movement and makes instantaneous adjustments to improve the match.9 The cerebellum receives information from proprioceptors in muscles and joints and from the vestibular apparatus in the inner ear about the position of the head in space. Some of the fastest-conducting neurons in the nervous system are involved in relaying sensory information to the cerebellum.
The cerebellar cortex is folded much as the cerebral cortex is folded, in a way that significantly increases surface area. Its tightly folded shape gives it a banded appearance. The cortical ridges on the surface of the cerebellum are called folia. The white matter beneath is called the medullary center and is made up of fibers running to and from the cerebellar cortex.
The cerebellum is divided anatomically, first by the posterolateral fissure, which separates the flocculonodular lobe (the region immediately inferior to the middle cerebellar peduncles) from the main body (Figure 43-14). The midline body is called the vermis, and it is straddled on either side by the cerebellar hemispheres.
The prominent tracts that attach the cerebellum to the brainstem are called the inferior, middle, and superior cerebellar peduncles. The inferior cerebellar peduncle is composed primarily of afferent fibers coming from the spinal cord and the brainstem. The middle peduncle contains afferent fibers from the contralateral pontine nuclei. The superior cerebellar peduncle is composed of major efferent pathways leaving the cerebellum.
Deep within the medullary center in each cerebellar hemisphere are the cerebellar nuclei. These include the dentate nuclei, the interposed nuclei, and the fastigial nuclei. The deep cerebellar nuclei are the final pathway of cerebellar output. Input from several areas of the cerebral cortex is received by the cerebellar hemispheres and dentate nuclei, and then sent back to the motor and premotor cortex. This circuit is believed to influence the planning and programming of voluntary movements, especially learned, skilled movements (those that become more rapid, precise, and automatic with practice).
The major input to the paravermal region, also called the intermediate cortex, consists of somatotopically arranged projections from the motor cortex and spinal cord. The intermediate cerebellum influences spinal cord and motor neurons through the corticospinal tract and the rubrospinal tract, where it is involved in interpreting and responding to the position and velocity of the moving body.
The vermis is most involved with regulation of posture and stereotyped movements that are programmed in the brainstem and spinal cord. The flocculonodular lobe helps maintain equilibrium and mediate the eye movements needed for visual tracking.
Lesions of the cerebellum result in ataxia (impaired balance), intention tremor, past pointing (failure of finger-to-nose test), and dysdiadochokinesia (failure of rapid movements).
Brainstem
The brainstem is a stalk of neural tissue that lies between the upper spinal cord and the diencephalon. It has three parts: from top to bottom these are the midbrain, pons, and medulla oblongata. The brainstem is critical for transmission of impulses between the brain and spinal cord. Vital centers for regulating respiratory and cardiovascular function are located in the medulla and pons. In addition, the reticular activating neurons that maintain consciousness and alertness traverse the brainstem to reach the thalamus. Of the 12 pairs of cranial nerves, 10 originate from nuclei in the brainstem; only cranial nerves I (olfactory) and II (optic) originate elsewhere (diencephalon).
The midbrain or mesencephalon contains the cerebral peduncles, consisting of motor tracts to the spinal cord; the superior and inferior colliculi, which control head and eye movements; and the red nucleus, part of a major motor tract. Cranial nerve III (oculomotor) emerges from the midbrain and is prone to compression when pressure in one of the cerebral hemispheres is elevated. Increased intracranial pressure (e.g., from tumor, ischemia, edema, bleeding) is commonly manifested by dysfunction of cranial nerve III resulting in abnormal pupil size and poor reactivity to light (see Chapter 44). Cranial nerve IV (trochlear) also emerges at the level of the midbrain.
The pons (Latin for bridge) connects the midbrain above to the medulla below. The dorsal pons consists of reticular formation fibers, ascending sensory tracts, and descending motor tracts. Two respiratory centers (pneumotaxic and apneustic) located in the dorsal pons work in coordination with the principal respiratory centers in the medulla. A major pathway of voluntary motor control, the corticospinal tract, also passes through the ventral pons on its way from the motor cortex to the spinal cord.
The medulla oblongata makes up the lower third of the brainstem and is continuous with the spinal cord. Nuclei within the reticular formation of the medulla form the vital centers that regulate cardiac, vascular, and respiratory function. The medulla also contains centers that coordinate swallowing, vomiting, coughing, and sneezing. The medulla is the site of decussation (crossing over) of the major sensory (dorsal column) and motor (corticospinal) tracts such that innervation of one side of the body is connected to the opposite (contralateral) cerebral hemisphere. The corticospinal tract neurons decussate within ridges on the ventral surface of the medulla called medullary pyramids. Motor tracts that do not cross over within the pyramids (e.g., tectospinal, vestibulospinal) are sometimes referred to as extrapyramidal tracts; disorders associated with function of these tracts (balance, posture, gait) may be called extrapyramidal disorders (e.g., Parkinson disease). Although the anatomic correlation is not quite accurate, use of the term persists in a clinical context.
All of the remaining cranial nerves (VI, VII, VIII, IX, X, XI, and XII) originate in the medulla. The cranial nerves themselves are part of the PNS and are discussed in that section. The name, origin, and function of the 12 cranial nerves are included in Table 43-4.
TABLE 43-4
CRANIAL NERVE | ORIGIN | FUNCTION |
I (olfactory) | Nasal mucous membrane | Olfaction |
II (optic) | Retina | Vision |
III (oculomotor) | Midbrain | Movement of eyeball, eyelid, constriction of pupil |
IV (trochlear) | Lower midbrain | Lateral eye movements |
V (trigeminal) Ophthalmic Maxillary Mandibular | Forehead, eyes Upper jaw, lip Lower jaw area | Sensation from forehead, eye, scalp Sensation from cheek, upper lip Sensation from chin and lower jaw, motor chewing |
VI (abducens) | Lower pons | Lateral eye movements |
VII (facial) | Pons | Taste from anterior tongue, control of muscles of face |
VIII (vestibulocochlear) | Cochlea Inner ear | Hearing Equilibrium |
IX (glossopharyngeal) | Medulla | Taste from posterior tongue, secretion of saliva, swallowing |
X (vagus) | Medulla | Monitors oxygen, carbon dioxide, and pH levels in blood; senses blood pressure; inhibits cardiac action and has extensive gastrointestinal activities |
XI (spinal accessory) | Medulla and cervical cord | Voice production, movement of head and shoulders |
XII (hypoglossal) | Medulla | Movements of tongue during speech and swallowing |
The Spinal Cord
The spinal cord conveys nervous impulses between the brain and 31 pairs of spinal nerves that innervate sensory organs and muscle cells of the body. The spinal cord mediates spinal reflexes involved in maintenance of posture, protective responses to pain, urination, and muscle tone. A great deal of integration and processing occurs in the gray matter of the spinal cord, whereas the white matter contains bundles of myelinated axons forming tracts that run up and down the cord. Tracts in the spinal cord are somatotopically organized such that the innervation of a particular body region is connected to a specific region in the cerebral cortex.
The typical adult spinal cord is about 18 inches long, extending from the base of the skull (foramen magnum) to the first or second lumbar vertebra (L1 to L2).10 The vertebral column extends for several more inches, providing a reservoir for CSF and exit points for the lumbar and sacral spinal nerves. The vertebral column is formed by interlocking sections of bone separated and cushioned by intervertebral disks. At the lateral aspect of the intersection of two vertebrae is an opening (intervertebral foramen) that provides a passageway for spinal nerves to exit the cord. The spinal cord travels in a small lumen (1 cm) in the center of the vertebral column and is itself only slightly larger than the diameter of a pencil10 (Figure 43-15).
On cross-section the spinal cord has a butterfly pattern of gray matter surrounded by white matter (Figure 43-16). Three bumps on the butterfly wings are called horns: the ventral horn (motor neurons), the dorsal horn (sensory neurons), and the lateral horn (sympathetic neurons). The horns consist of neuron cell bodies, synapses, and small unmyelinated interneurons. The white matter is divided into columns that contain tracts of nerve fibers traveling to and from the brain. These are the posterior (dorsal) columns, anterior columns, and lateral columns. Some of the neurons in the columns convey signals from one level of the cord to another and are important in reflex and postural adjustments. The principal ascending sensory tracts include the dorsal column–lemniscal and the anterolateral (spinothalamic) tracts, which send afferent signals to the brain. The principal descending motor tracts include the corticospinal, rubrospinal, reticulospinal, and vestibulospinal tracts. These tracts are located in specific regions of the cord (Figure 43-17). Sensory and motor pathways are discussed in later sections of this chapter.
Spinal nerves divide into two sections as they make contact with the spinal cord: the ventral and dorsal roots (Figure 43-18). Ventral roots contain motor neurons that originate in the anterior horn and travel in the spinal nerve to skeletal muscles. Dorsal roots carry sensory information from somatic receptors to neurons in the posterior horn. The cell bodies of sensory afferents collect together in the dorsal root ganglion. Autonomic nerves also travel in the spinal cord and exit and enter the cord by way of the ventral and dorsal roots.
The points at which sensory neurons enter the cord and at which motor neurons exit represent the separation of the CNS and PNS.
Peripheral Nervous System
The PNS consists of the 31 pairs of spinal nerves and the 12 pairs of cranial nerves. These nerves are myelinated with Schwann cells, which differ somewhat from the oligodendrocytes that form the myelin sheaths of CNS neurons. By convention, groups of cell bodies are called ganglia in the PNS and nuclei in the CNS. A major exception to this naming rule is the basal ganglia of the CNS. The PNS is not protected by CSF, meninges, or bony coverings as is the CNS; however, a sheath of connective tissue covers the nerves and provides support.
The PNS serves both afferent sensory functions and efferent motor functions of the somatic and autonomic systems. Cranial nerves III, VII, IX, and X and spinal nerves S2 and S3 contain parasympathetic neurons, and spinal nerves T1 to L2 contain sympathetic neurons.11
Cranial Nerves
As previously noted, all of the cranial nerves originate in the brainstem except cranial nerves I and II, which originate in the diencephalon12 (Figure 43-19). Cranial nerve I is strictly sensory, transmitting olfactory signals from the 10 million to 20 million olfactory neurons in the nasal cavities to the olfactory bulbs. The olfactory bulb neurons then project to the olfactory cortex. Cranial nerve II is also sensory, conveying visual information from the retina to the brain. The optic nerve is unusual in that it is an extension of the CNS, myelinated by oligodendrocytes rather than Schwann cells. The neurons from the medial retina decussate in the optic chiasm, whereas the lateral retina neurons do not. Thus, the right visual field projects to the left hemisphere and the left visual field projects to the right hemisphere. Damage to one hemisphere, as occurs in stroke, often interrupts visual signals from the corresponding sides of each retina—a condition known as homonymous hemianopsia (see Chapters 44 and 46).
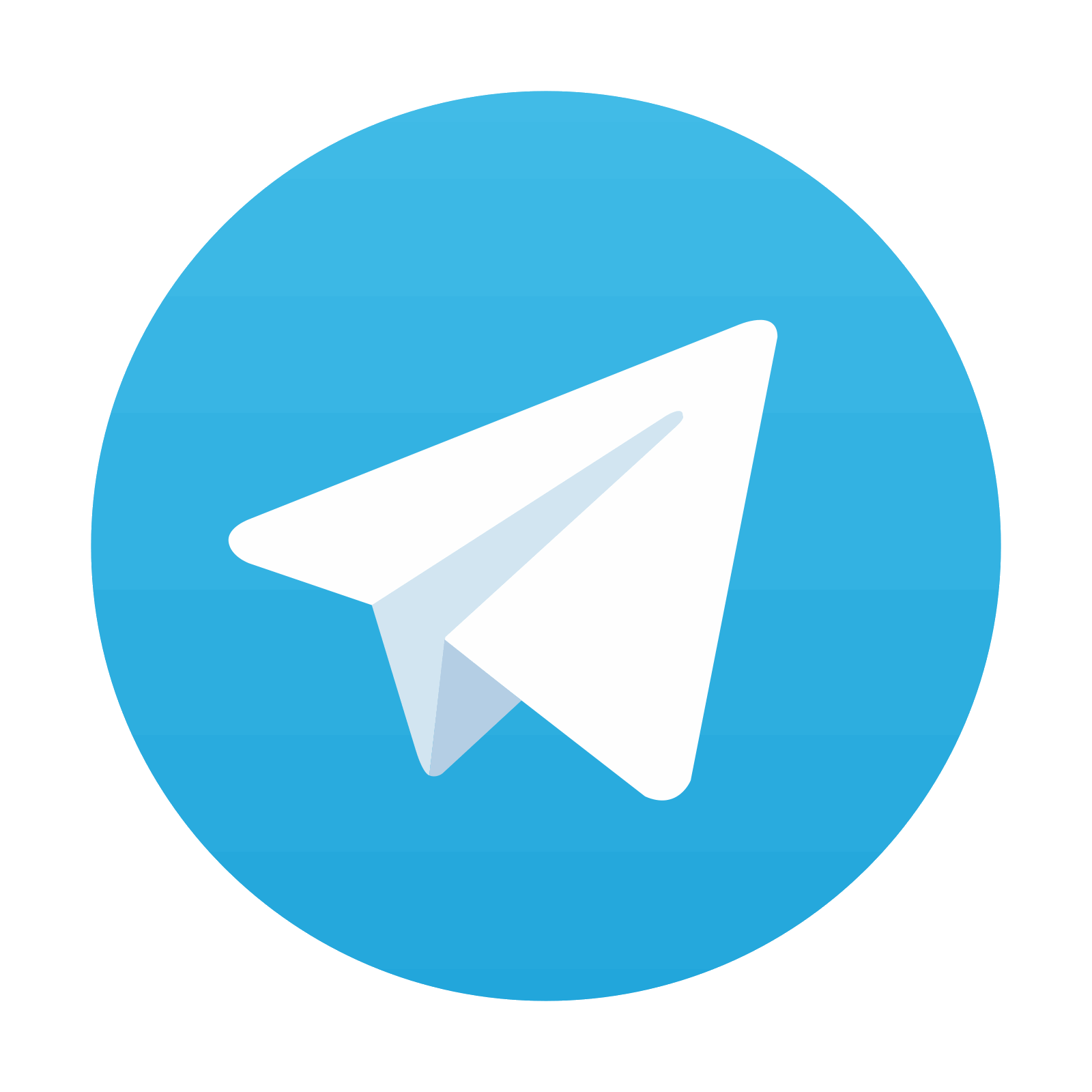
Stay updated, free articles. Join our Telegram channel
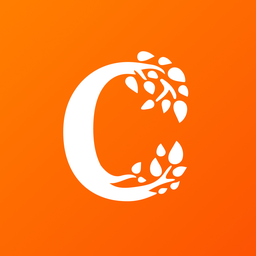
Full access? Get Clinical Tree
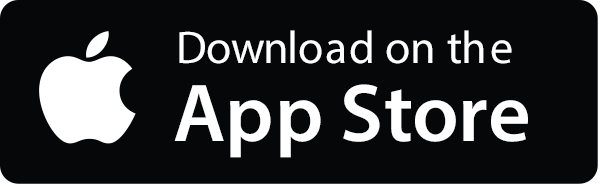
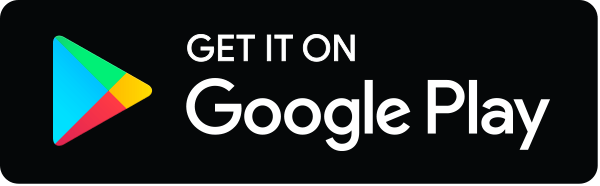