Alterations in Blood Pressure
Robin Beeman
Key Questions
• How do changes in cardiac output and systemic vascular resistance affect blood pressure?
• How is blood pressure regulated on a short- and long-term basis?
• What are the risk factors for the development of primary hypertension?
• How is secondary hypertension defined, and what are the common etiologies?
• How is hypertension detected, classified, and managed?
• What are the end-organ consequences of inadequately controlled hypertension?
• What are the risk factors for orthostatic hypotension, and how is the condition managed?
http://evolve.elsevier.com/Copstead/
Meeting the needs of the body’s tissues for oxygen and nutrients requires both adequate blood flow at the tissue level and sufficient perfusion pressure systemically to force that blood forward. The systemic arterial blood pressure provides that momentum, and the tissues depend on its preservation to ensure their metabolic needs are met. This maintenance requires a complex regulatory system. The body’s organs can be damaged if the perfusion pressure is insufficient or if it is excessive.
Arterial Blood Pressure
As described in Chapter 15, oxygenated blood is propelled from the left side of the heart into the arterial circulatory system, and following a pressure gradient, travels to the capillary beds of the body’s tissues (Figure 16-1). There, oxygen and nutrients are exchanged for metabolic wastes, and the blood then returns to the right side of the heart via the venous circulatory system, where it passes through the lungs to repeat the process. It is the pressure difference between the left and right sides of the heart that produces the gradient allowing this systemic movement of blood. The arterial blood pressure is produced by the force of left ventricular contraction overcoming the resistance of the aorta to open the aortic valve, and is the pressure maintained in the arterial system throughout the cardiac cycle.
Determinants of Systemic Blood Pressure
The systemic arterial blood pressure is the physiologic result of the cardiac output and the resistance to the ejection of blood from the heart.1 Cardiac output (CO) is the product of two variables: stroke volume (SV) and heart rate (HR) (CO = SV × HR). SV is the specific volume of blood leaving the heart with each contraction, which itself is determined by the volume of blood in the heart before systole (end-diastolic volume) and the contractility of the myocardium.1 The end-diastolic volume is determined by the amount of blood returned to the heart between contractions, and is typically called the heart’s preload. Stroke volume multiplied by the number of contractions of the heart per minute (heart rate) determines the amount of blood leaving the heart—the cardiac output, measured in liters per minute. The resistance to ejection into the arterial circulation is known as the systemic vascular resistance (SVR) and is determined by the radius of arteries and the degree of vessel compliance. SVR is synonymous with cardiac afterload, and can be altered by constricting or relaxing (dilating) arterial smooth muscle. It can be calculated by using a derivation of Poiseuille’s law (see Chapter 15). This physical law states that in a tube with laminar flow, resistance is primarily determined by three factors: the radius of the tube, the length of the tube, and the viscosity of the fluid. Applied to SVR, because the viscosity of the blood and the total length of the arterial system are normally relatively constant, the radius of the arterioles becomes the major determinant of resistance. Therefore, the formula for blood pressure is: BP = CO × SVR. Alteration in any one of these variables will result in a change in blood pressure. This basic concept is important to normal physiologic function, disorders of blood pressure, and the therapeutic interventions undertaken to treat them. The pulmonary vascular bed contributes minimally to total systemic resistance and is seen as a separate resistance system, called pulmonary vascular resistance (PVR). It has its own pathology discussed in Chapter 21.
Measurement of Blood Pressure
Components of Blood Pressure Measurement
Arterial blood pressure is measured from its highest point during cardiac systole to its lowest during diastole. These are referred to as systolic pressure and diastolic pressure, respectively, and are measured in millimeters of mercury (mm Hg). During ventricular contraction, the pressure in the aorta rises to an average peak value of approximately 110 mm Hg in the adult1 (see Figure 16-1). Whatever this peak pressure may be, it is referred to as the systolic blood pressure. The smooth muscle of the aorta passively recoils from this point, ejecting blood forward into the peripheral arteries at that given pressure. Stroke volume is the primary factor impacting systolic pressure; an increase or decrease in SV produces a corresponding change in systolic blood pressure. During ventricular diastole, the pressure in the arterial system falls to an average minimum value of 70 mm Hg in the adult. The value of this minimum pressure is called the diastolic blood pressure. SVR is the major determinant of diastolic blood pressure; an increase or decrease in diastolic pressure is the result of a corresponding increase or decrease in arterial resistance (SVR). The difference between systolic and diastolic blood pressure is termed the pulse pressure.1 Therefore, the pulse pressure for a systolic pressure of 110 mm Hg and a diastolic pressure of 70 mm Hg would be 40 mm Hg.
Systolic and diastolic values are normed by age. Standards for the identification of normal blood pressure and levels of abnormal elevation have been established. The most precise standards for children are those based on height, age, and gender2,3 (Table 16-1). Standards for blood pressure have likewise been determined for the adult4 (Table 16-2).
TABLE 16-1
BLOOD PRESSURE CLASSIFICATION IN CHILDREN AND ADOLESCENTS
SBP and DBP <90th percentile∗ | Normal for children and adolescents |
Average SBP and/or DBP ≥90th percentile but <95th percentile† | Prehypertension in children or adolescents |
SBP ≥120 and/or DBP ≥80 mm Hg | Prehypertension in adolescents‡ |
Average SBP and/or DBP ≥95th percentile† | Hypertension in children and adolescents |
DBP, Diastolic blood pressure; SBP, systolic blood pressure.
†For age, height, and gender measured on at least three separate occasions.
‡The same criteria used for prehypertension in adults are also applied to adolescents.
Selected data from the Fourth Report on the Diagnosis, Evaluation, and Treatment of High Blood Pressure in Children and Adolescents, Pediatrics 114(2):555-576, 2004.
TABLE 16-2
BLOOD PRESSURE CLASSIFICATION IN ADULTS
CATEGORY | SBP (mm Hg)∗ | DBP (mm Hg)∗ |
Normal | <120 | <80 |
Prehypertension | 120-139 | 80-89 |
Stage 1 hypertension | 140-159 | 90-99 |
Stage 2 hypertension | ≥160 | ≥100 |
DBP, Diastolic blood pressure; SBP, systolic blood pressure.
∗Classification determined by the higher value.
From Chobanian AV: Seventh Report of the Joint National Committee on Prevention, Detection, Evaluation, and Treatment of High Blood Pressure: The JNC 7 Complete Report, Hypertension 42:1206-1252, 2003.
Mean arterial pressure (MAP) is the calculated average pressure within the circulatory system throughout the cardiac cycle. Because more time is spent in diastole than in systole, MAP is not the arithmetic average of diastolic and systolic pressure but rather reflects the relative time spent in each portion of the cardiac cycle.5 The calculation may be performed by computer during direct arterial blood pressure measurement, as described below, but is most conveniently determined by a simple formula using the values of blood pressure obtained indirectly. Several formulas are available, and they may use systolic, diastolic, or pulse pressures; the most common formula uses the systolic and diastolic pressures as follows:

For a person with a systolic pressure of 110 mm Hg and a diastolic pressure of 70 mm Hg, the MAP would be:

MAP is used clinically as part of cardiovascular assessment and in the incremental adjustment (titration) of parenterally administered vasoactive drugs.
Direct Measurement of Blood Pressure
Direct measurement of blood pressure is one aspect of hemodynamic monitoring and requires an intraarterial catheter and specialized equipment to transduce the arterial fluid pulsations into electrical signals. The catheter most often is placed in the radial artery. These signals are then displayed on a computer screen as waveforms, and the systolic, diastolic, and MAPs are digitally represented. This is the most accurate method of measuring blood pressure available, but is typically only performed in controlled settings, such as surgical or critical care units, and carries its own risk of measurement error.5 A detailed discussion of hemodynamic monitoring is beyond the scope of this text.
Indirect Measurement of Blood Pressure
Blood pressure is most commonly measured by indirect means at the brachial artery, using a mercury sphygmomanometer and a stethoscope for auscultation or an automated oscillometric system such as Dinamap® or the Welch Allyn Spot Vital Signs®. Wrist or finger monitors are not recommended because of the inaccuracy of the values obtained compared with brachial measurements.6 Specific, evidence-based standards are available for the correct use of these noninvasive automated systems for adults and children including scheduled calibration,6–8 and they are inherently less accurate if the blood pressure is significantly increased or decreased, or if there are cardiac dysrhythmias.8 Because the values in blood pressure references are based on the auscultatory method, and it is the easiest method and least stressful to patients, it is the preferred measurement technique.7,9 Although the brachial artery is typically used for convenience, certain assessment procedures require recording the blood pressure at other arterial sites (e.g., ankle-brachial index). Several studies have reported differences between the right and left arm pressures, but no pattern of differences is evident.6 Other studies report that in the absence of disease, systolic pressures do not differ significantly at a clinical or statistical level between the right and left arms.10 In practice, it is recommended that blood pressures be initially taken in both arms and the arm with the highest value be recorded.6 In situations such as a shock state, when systolic and diastolic pressures cannot be auscultated, the systolic pressure alone may be obtained by palpation or by amplification of the pulse using ultrasound technology (Doppler pressure).
Auscultated and oscillometric blood pressure measurements are burdened with the potential of measurement error, in both reliability and validity (Table 16-3). This dictates the need for careful technique, and in most cases enhances the value of trend data as opposed to individual readings. The individual patient’s heart rate, degree of arterial compliance, and dynamics of blood flow may vary over time. Inappropriate blood pressure cuff size, arm position, and both the visual and auditory acuity of the clinician may impact the accuracy of individual readings.9 An additional source of error has been named the “white coat effect” for the elevation of blood pressure when taken in a clinic or office environment.3,11 First described in 1897 by Scipione Riva-Rocci, who was the first to document assessing the systolic pressure by palpating the brachial artery,12 these situational elevations in blood pressure are of concern because treatment may be initiated based on inaccurate data.11 This condition is most common in older individuals of either gender, but may occur at any age.6 Pickering and colleagues report that in approximately 15% to 20% of patients with stage I hypertension, elevated blood pressure may only be persistent under these circumstances. Significant pressure differences have been found using the automatic noninvasive technology between the supine, 45-degree elevation of the head of the bed, and sitting position in the same patient,13 and between multiple body positions using the auscultatory method.6,14 Normal values are based on the subject being seated, with the back supported and the arm at heart level. Specific recommendations regarding all aspects of indirect measurement are provided by the American Heart Association,6 and sources of error within pediatric populations also have been documented.15
TABLE 16-3
INTRINSIC AND EXTRINSIC FACTORS THAT INFLUENCE INDIRECT BLOOD PRESSURE ACCURACY
The recommended approach for obtaining an auscultated blood pressure is a two-step approach, beginning with inflating the cuff to the point at which the pressure obliterates the palpated radial pulse (systolic pressure). The pressure is completely released and after 15 to 30 seconds the cuff is reinflated to 30 mm Hg above that point, and then gradually deflated while the clinician listens through the stethoscope with the diaphragm placed over the brachial artery and monitors the position of the mercury in the sphygmomanometer. The return of blood flow through the artery is signaled by the sounds produced by the turbulent flow through the partially occluded artery and named after the Russian physician who first described them in 1905 (Korotkoff sounds).12 This sound is recorded as the systolic pressure. As the pressure continues to be released, sounds change in intensity until the point at which the Korotkoff sounds disappear, which is noted as the diastolic pressure (Table 16-4). Nurse researchers in Britain found statistically significantly lower diastolic values using this approach compared with a one-step approach.9 In this approach, the systolic pressure was estimated by palpating the brachial artery during cuff inflation, and inflation continued 30 mm Hg beyond that point before proceeding with deflation. It was postulated that the first inflation and occlusion produced a reactive vasodilation that could be responsible for this difference.9 Regardless, the auscultation of Korotkoff sounds results in systolic values that are lower than those obtained by direct, intraarterial blood pressure measurement.6 Older patients often have a period during measurement when the Korotkoff sounds disappear, returning 20 to 40 mm Hg later. This auscultatory gap may be attributed to intraarterial pressure fluctuations associated with hypertension (Figure 16-2) and can often be eliminated by elevating the arm above the level of the head for 30 seconds before cuff inflation. This approach is postulated to enhance the audibility of Korotkoff sounds by increasing arterial flow following the increase in venous return.6
TABLE 16-4
PHASE | DESCRIPTION |
I | Initiation of clear tapping sounds—systolic blood pressure |
II | Murmuring or swishing sounds |
III | Increase in intensity and crispness of sounds |
IV | Muffling of sounds |
V | Disappearance of sounds—diastolic blood pressure |
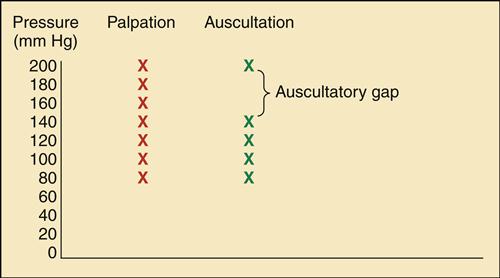
Palpating the blood pressure (BP) before auscultation allows assessment of the true systolic BP. Palpated BP equals 200/P. The same result can often be obtained by elevating the arm overhead for 30 seconds before inflating the cuff. Auscultated BP when the cuff is inflated to only 180 mm Hg results in a falsely low value of 140/80 mm Hg.
Increasingly, self-monitoring of blood pressure is being performed at home. Potential sources of error as well as optimal schemes of measuring and recording have been identified.16–18 It has been found that the values documented in this setting are more accurate, if correctly obtained, because of the elimination of the white coat effect.3,11,18
Mechanisms of Blood Pressure Regulation
Arterial blood pressure is physiologically controlled on both a short-term and a long-term basis. Regulation of blood pressure is achieved through changes in factors that impact the primary determinants of blood pressure: heart rate, stroke volume, and SVR (Figure 16-3). These variables are affected by a complex interplay between neural, humoral, and renal factors to maintain stability in the face of ever-changing internal and external environmental demands. An understanding of these mechanisms is essential to exploring pathophysiologic alterations. Blood pressure normally fluctuates over the course of 24 hours owing to physiologic changes associated with circadian rhythm.
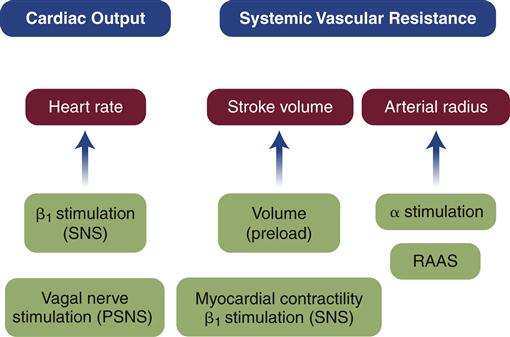
Some of these provide short-term adjustments, whereas others affect the long-term management of blood pressure. PSNS, Parasympathetic nervous system; RAAS, renin-angiotensin-aldosterone system; SNS, sympathetic nervous system.
Short-term Regulation of Systemic Blood Pressure
Changes in blood pressure must occur quickly to accommodate behavioral changes (e.g., position changes, exercise), emotional changes (e.g., fear, anxiety), and physiologic changes (e.g., fever, volume depletion). Changes in physical activity require the most frequent alterations, and rapid adjustments are initiated in seconds so that the arterial blood pressure may be increased to twice the normal value within 5 to 10 seconds.1 This short-term regulation is mediated by the sympathetic branch of the autonomic nervous system (the sympathetic nervous system, SNS). Activation of the SNS influences both heart rate and SVR. The force of contraction is primarily a factor of the circulating volume (preload) and affects long-term regulation of arterial blood pressure.
Modifications in systemic blood pressure are made by activation of the SNS directly or indirectly through stimulation of the baroreceptor reflex. (Autoregulatory changes in pressure at a local level, at the tissues of body organs, are discussed in more detail in Chapter 15.) These SNS activities related to the distribution and pressure of blood are directed through the vasomotor center in the medulla of the brainstem while the lower centers of the brain monitor the body’s internal and external environments.1 The vasomotor center is directly activated by such stimuli as fever or external stressors to evoke increased activity and elevate systemic arterial blood pressure.
The autonomic nervous system maintains a basal level of arteriolar smooth muscle tone through the SNS, and provides heart rate control through a balance of SNS and parasympathetic nervous system (PSNS) activity. Stimulation of the SNS results in the increased release of the neurotransmitters epinephrine and norepinephrine. At the smooth muscle of the arterial system, these neurotransmitters bind to α1 receptors to initiate vasoconstriction and an increase in SVR. Stimulation of the PSNS has almost no effect on most systemic vessels, other than venodilation in localized areas such as the face, producing a blush.1 Receptors within the brain (α2) provide negative feedback regulation, decreasing the central release of epinephrine and norepinephrine in response to stimulation. In the heart, the binding of these neurotransmitters to β1 receptors results in an increase in the rate of firing at the sinoatrial (SA) node, increasing the heart rate in response to increased demands. The PSNS is responsible for maintaining a slower heart rate during periods of rest.1
Indirectly, the vasomotor center is stimulated by a decreased rate of discharge by baroreceptors. Pressure-sensitive receptors (baroreceptors) are found in the vessel walls of nearly all large arteries in the thorax and neck, but are particularly plentiful in the sinuses of the carotid arteries and in the arch of the aorta.1 Signals from the aorta travel through cranial nerve X and those from the carotids are transmitted through cranial nerve IX; both terminate in the vasomotor center of the medulla. These specialized receptors are sensitive to changes in MAP. They transmit impulses continuously, altering their rate of discharge in response to changes in MAP. Their response to these changes is very brisk, especially when pressure changes occur rapidly, which makes them the perfect mechanism to respond to variations in body position and minimize the gravity-induced decreases in pressure in the upper body.1 A decrease in sensed pressure induces a decrease in action potential formation by the baroreceptors. This causes the vasomotor center to increase SNS outflow to the heart and arterial bed and to decrease PSNS stimulation to the heart. The net result is an increase in both heart rate and SVR, producing an increase in blood pressure. An increase in sensed pressure results in an increased rate of firing by the baroreceptors and a negative feedback response, lowering systemic arterial pressure. The responsiveness of the baroreflex declines with age; age-related stiffening of the arterial walls has been implicated along with contributions from pathologic conditions such as hypertension and diabetes mellitus, which are more common in the older population.1,19 The results of animal studies indicate that the overall effect of the baroreflex is a reduction of the minute-to-minute fluctuations in arterial blood pressure by 33% of what it would be without this mechanism.1 There is abundant evidence that within 1 to 2 days of exposure to chronic elevations of blood pressure, baroreceptors reset to the new level and the rate of discharge begins to decrease and then slowly returns to the norm despite an elevated baseline pressure.11,19 This finding suggests that the baroreflex may contribute to long-term blood pressure regulation through the SNS stimulation of the kidneys1 discussed in the next section.
Receptors in the carotid and aortic arterials respond to chemical signals of hypoxia (H+ and CO2 level elevations) that occur when arterial pressure declines. These chemoreceptors stimulate the medullary vasomotor center to increase SNS activity. However, this mechanism responds significantly only when systolic pressures decrease below 80 mm Hg, so blood pressure can be prevented from falling even lower.20
Long-term Regulation of Systemic Blood Pressure
The regulation of arterial blood pressure on a long-term basis, week after week and month after month, is accomplished through the interplay of neural, hormonal and renal interaction21,22 and is intimately connected with the body’s fluid volume homeostasis.1 The balance of the intake of water and sodium with their excretion by the kidney remains the central feature of long-term blood pressure maintenance. Historically, the role of the renin-angiotensin-aldosterone system (RAAS) has been seen as the primary contributor to this process, and although it continues to be a major determinant, mechanisms involving the baroreflex and the vasomotor center in the brainstem as well as localized renal systems are receiving increased attention in research.1,21,22
An increase in extracellular fluid (ECF) volume, because of increased intake or decreased excretion, results in an increase in cardiac output; when combined with the volume-induced increase in systemic vascular resistance, this results in an elevation in the arterial blood pressure (Figure 16-4). Body tissues initiate their local autoregulation mechanisms, constricting arterioles to protect against high-flow damage, which further contributes to the overall arterial resistance in the body. Unless fluid intake or renal functions are abnormal, this increase in systemic vascular resistance will not result in a prolonged elevation in arterial pressure.1 The kidneys will respond quickly, increasing excretion of sodium and water and normalizing pressure within a matter of hours. This physiologic regulatory response may be disrupted if the renal vasculature is constricted, as occurs in hypertension.1
Because sodium is not as rapidly eliminated by the kidney as water, elevations in sodium intake are more likely to elevate arterial pressure.1 Excess sodium also adds to the body’s fluid volume by several mechanisms. Sodium increases the osmolality of the ECF and activates the central thirst center, causing an increase in water intake. The increased serum osmolality will be sensed by the hypothalamus and posterior pituitary, causing the release of antidiuretic hormone (ADH) into the bloodstream. Once ADH arrives in the renal vasculature, it binds to receptors in the collecting ducts, resulting in the enhanced reabsorption of water in order to decrease osmolality (Chapter 26).
The physiologic mechanisms of the RAAS are tightly controlled and interdependent (Figure 16-5). Prorenin, the inactive form of renin, is synthesized and stored by specialized smooth muscle cells located in the afferent arterioles of the kidney situated immediately proximal to the glomeruli.1 Known as the juxtaglomerular cells, these cells are stimulated by a decrease in arterial pressure to enzymatically cleave the precursor, and release the activated renin enzyme into the vascular bed of the kidney. Most of the renin travels into the general circulation where it acts on a circulating plasma protein called angiotensinogen, resulting in the release of angiotensin I, a peptide possessing minimal vasoconstrictive capacity.1 Angiotensin I continues to be created by renin for about 30 to 60 minutes, until renin is removed from the body. While the blood carrying angiotensin I circulates through the pulmonary vessels, an enzyme produced by the vascular endothelium (angiotensin-converting enzyme [ACE]) comes in contact with angiotensin I, and two amino acids are fragmented from angiotensin I to produce angiotensin II.1 Inactivated in minutes by angiotensinases, continued production of angiotensin II maintains the profound effects it initiates. Angiotensin II is an extremely potent vasoconstrictor, primarily of the arterial bed but also slightly affecting the venous system. The SVR is therefore increased, raising blood pressure. The vasoconstrictive response to angiotensin II requires about 20 minutes to reach maximal capacity, but is capable of elevating arterial pressure to 50% of normal after severe hemorrhage.1 The enhanced venous return attributable to the elevated SVR improves cardiac function by increasing myocardial fiber stretch, producing increased contractility and therefore stroke volume. Angiotensin II also is an intermediary for an additional means of raising blood pressure—increasing circulating volume to significantly increase venous return to the heart and therefore stroke volume. Angiotensin II in the general circulation reaches the cortex of the adrenal glands, stimulating the release of the hormone aldosterone. Aldosterone circulates to the kidneys where it binds to receptors in the renal tubules, causing the kidneys to reabsorb more sodium. Water follows the sodium back into the bloodstream. The result is an increase in blood volume and further elevation in blood pressure. Excessive amounts of angiotensin in the bloodstream have been found to effectively reset this mechanism of blood pressure control to a higher-than-normal level,1 potentially contributing to hypertension.
Some of the renin released by the juxtaglomerular cells exerts local effects within the kidney to elevate blood pressure.1,23 Renin receptors in the mesangium of the glomerulus and below the endothelial cells of the renal arteries are activated to enhance the conversion of angiotensinogen to angiotensin I.23 Angiotensinogen has been isolated in tubular and mesangial cells, and angiotensin-converting enzyme is found both in vascular endothelium and in the epithelium of the tubular cells.1,23
Other influences on long-term arterial blood pressure control include the activity of the SNS, levels of natriuretic peptides, and regulation of intrarenal mechanisms such as renal medullary endothelin production.1,23 Renin release is increased when neurotransmitters released by the sympathetic nervous system bind to β1 receptors in the kidney. Additional local SNS effects include decreased glomerular filtration rate as a result of renal arteriolar constriction and increased tubular reabsorption of sodium and water caused by increased quantities of angiotensin II and aldosterone.1,23 These effects contribute to the increased systemic blood pressure associated with severe prolonged stress. Increased SNS activity has been documented to be present in hypertension, and its role is confirmed because antihypertensive drugs that affect autonomic control of heart rate and systemic vascular resistance are so clearly effective in treatment.1 A number of natriuretic hormones play a role in arterial pressure through their effects on ECF volume regulation; most important of these is atrial natriuretic peptide (ANP).1 Increased volume in the atria of the heart triggers stretch receptors and stimulates the release of ANP into the bloodstream by cardiac muscle fibers. ANP causes the kidneys to increase water and sodium excretion by increasing glomerular filtration rate (GFR) and decreasing sodium reabsorption so both sodium and water remain in the filtrate.1 This diuretic effect reduces circulating volume and therefore blood pressure. Endothelin-1 (ET-1) is a peptide produced in the renal medulla.22 ET-1 binds to receptors within the kidney, initiating an autocrine-induced vasodilatory response affecting renal perfusion, water and electrolyte movement, and release of renin. This makes ET-1 an important participant in normal systemic blood pressure control, and levels have been found to be decreased in hypertension.22 Most likely, long-term blood pressure control is a reflection of the unified contributions of all the factors discussed here, and more are yet to be identified.
Normal Fluctuations in Systemic Blood Pressure
Many homeostatic mechanisms of the body undergo daily variations in their function governed by an area of the brain called the suprachiasmatic nuclei—the body’s internal clock. Brain wave activity, cell regeneration, cortisol release, body temperature, heart rate, and blood pressure are only a few of the more than 100 circadian rhythms.24 In the case of blood pressure, it is known that it rises before awakening (morning surge), is highest in the middle of the morning, then begins to fall, and reaches its lowest level at night (nocturnal dip).25 In their recent review of the available research, Peixoto and White25 found these basic fluctuations to be primarily determined by internal neural and hormonal regulation, as well as by external environmental factors such as sodium intake and physical activity. Additional factors known to impact the normal rhythmic changes in blood pressure include lifestyle influences such as alcohol consumption and cigarette smoking, as well as cognitive activity and emotional state. Elevated blood pressure levels at specific points within the circadian rhythm have been documented to be associated with the development of diabetic nephropathy and of cardiovascular conditions such as an increased incidence of sudden cardiac death, myocardial infarction, and unstable angina, as well as stroke.25