Structure and Function of the Musculoskeletal System
Carol L. Danning
Key Questions
• What are the functions of osteoblasts and osteoclasts in bone remodeling?
• What is the relationship between joint structure and joint mobility?
• Why is articular cartilage particularly susceptible to degenerative changes?
• What factors determine tendon strength and compliance?
• How does the striated structure of skeletal muscle relate to its contractile function?
http://evolve.elsevier.com/Copstead/
Movement is one of the most characteristic and visible aspects of human life. Ease of movement adds to self-worth and well-being because the ability to move is closely connected to independence. A working knowledge of the system responsible for body movement is imperative to the health care provider. This chapter examines the basic characteristics of the firm support of bone and joint structures that make motion possible and the properties of skeletal muscles that are responsible for actually moving the body’s framework.
Structure and Function of Bone
The primary purposes of the skeletal system are to protect internal organs, provide bony attachments for muscles and ligaments, present rigid levers to allow functional movement of the body and its separate parts, and store mineral and marrow elements for forming new blood cells. Bone is highly vascular and is metabolically active from birth to death.
Composition
Bone is comprised of three main components: an organic matrix, an inorganic mineral content, and water. Accounting for approximately 25% of bone weight is an organic matrix (called osteoid) that is composed mostly of collagen fibers (about 94% of matrix). These collagen fibers, extending along lines of tension, give bone its tensile strength and some flexibility. Also part of the organic matrix is a homogeneous ground substance composed of protein polysaccharides, particularly proteoglycan, which binds between collagen fibers. These proteins may serve to transfer mechanical information within the matrix to bone cells.1
The cellular component of the organic matrix includes predominantly osteoblasts, osteocytes, and osteoclasts. Osteoblasts, formed from osteoprogenitor cells that line bone surfaces, produce the organic matrix (osteoid), which is subsequently mineralized to form new bone. Osteoblasts communicate with each other in a network of cell extensions to exchange minerals, nutrients, and stimulatory signals. When an osteoblast becomes engulfed in its own calcified matrix, it becomes a mature osteocyte within the bone but is still connected with other cells via its extended cell processes. Osteoclasts migrate to the bone surfaces in response to certain stimuli and are responsible for bone resorption and mobilization of minerals.
The second and largest component of bone is the inorganic material (mineral salts such as calcium and phosphate), which accounts for approximately 70% of bone weight. This mineral content, bound and embedded within the matrix mostly in crystals of calcium hydroxyapatite, gives bone its hard, rigid structural strength while also serving as the body’s main reservoir for calcium and phosphorus.1
Approximately 5% of bone weight is from water located within the organic matrix surrounding collagen fibers and ground substance and within the canals that carry nutrition to bone tissues.
Microscopically, the basic unit of bone is the osteon or the haversian system (Figure 50-1). The haversian canal lies at the center of each osteon and contains blood vessels and nerve fibers. A concentric series of lamellae of mineralized matrix surrounds the central canal. Bordering the lamellae are small cavities (lacunae) that contain a bone cell, the osteocyte. Many small channels, the canaliculi, connect adjacent lamellae with each other and eventually with the main haversian canal. This canal system allows nutrients from blood vessels in the haversian canal to reach osteocytes. Collagen fibers connect one lamella to another within the osteon and increase the mechanical strength of bone.2
At the tissue level, bones are classified as two types: cancellous or trabecular bone and compact or cortical bone (Figure 50-2). Cancellous bone is formed in thin plates called trabeculae found within the center of long bones, vertebral bodies, and flat bones such as the pelvis. Trabeculae are laid down in response to stress and are shaped to accommodate loads placed on the bone. Cancellous bone is covered by compact bone. Compact bone is quite resistant to compression and is dense in structure. Compact bone is laid down in concentric layers. A tough fibrous membrane called the periosteum covers all bones. The periosteum is highly vascularized and provides nutrition for bone via Volkmann canals (see Figure 50-1). An inner layer of the periosteum contains osteoblasts, which are responsible for bone growth and repair. The periosteum covers the entire bone except for the ends, which are covered by hyaline cartilage.
In longer bones, a central cavity (medullary cavity) is present (see Figure 50-2). A thin membrane called the endosteum covers this cavity. The central cavity is filled with fatty marrow. Osteogenic cells are located in the endosteum.
Blood vessels are distributed through the haversian canals. Living cells in bone communicate with each other and the haversian system via threadlike processes.
Functional Properties
Growth and Ossification
The process of longitudinal bone growth involves endochondral ossification. In this type of growth, cartilage is replaced by bone; this process of growth is evident in embryonic development, fracture healing, and some bone tumor growth. In children, fracture through the shaft of a long bone stimulates bone growth, possibly because of increased nutrition to growth cartilage from the hyperemia associated with fracture healing. Particular attention to bone alignment or overlapping of fracture ends must be paid when managing a fracture in a child younger than 10 years of age because bone overgrowth or skeletal arrest can lead to limb length discrepancies.3
Circumferential bone growth occurs via intramembranous ossification. In this case, connective tissue is transformed into bone. Interstitial growth is not possible in bone. Bone can grow in length only by a process of growth within cartilage, followed by endochondral ossification (Figure 50-3). Two sites of cartilage growth are available in a long bone: articular cartilage and epiphyseal plate cartilage. In longer bones, the epiphysis provides the only growth plate for the entire bone.
Continuous Growth
The epiphyseal plate (see Figure 50-3) allows for lengthening of the metaphysis and diaphysis of a long bone and is the site of continuous growth. Growth and thickening of cartilage cells of the plate move the epiphysis away from the metaphysis. Calcification and replacement of cartilage occur on the metaphyseal surface (endochondral ossification). Injuries to this growth plate in children may lead to limb length discrepancies. In addition, inflammatory arthritis in children (particularly of the knee) can lead to increased blood flow to the epiphyseal plate, which also may accelerate growth or lead to premature plate closure. This also can lead to leg length abnormalities.
The function of the epiphyseal plate in the growth process may be illustrated by examining the specific zones of the plate (Figure 50-4) and determining how they contribute to the growth process. The zone of resting cartilage maintains adherence of the plate to the epiphysis. Immature chondrocytes and vessels penetrate this first zone from the epiphysis and nourish the plate. The zone of young proliferating cartilage demonstrates the most active cartilage cell growth. The zone of maturing cartilage contains the enlarged and mature cartilage cells as they migrate toward the metaphysis. The final zone is the zone of calcifying cartilage, which is a very thin line of chondrocytes and the weakest segment of the epiphyseal plate. These chondrocytes are no longer living because of calcification of the matrix.
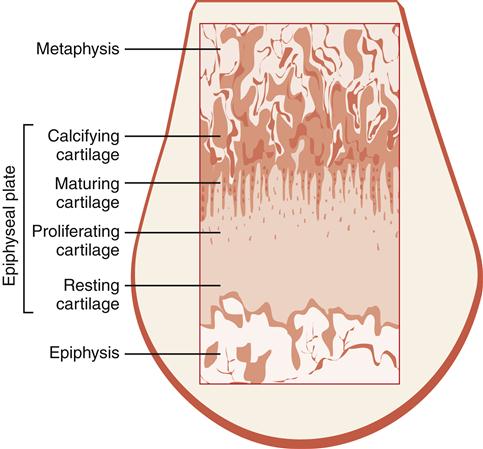
Bone is also deposited quite actively on the metaphyseal side of the plate. With the addition of new bone, the metaphysis becomes longer.
Osteoblasts in the inner layer of the periosteum are responsible for growth in the width of bones. This process is called intermembranous ossification. Resorption of bone, through a process of osteoclastic resorption, causes the medullary cavity to enlarge, causing additional widening of bone.
Hormones influence bone growth. Inadequate secretion of thyroxine by the thyroid gland or insufficient growth hormone secretion from the pituitary gland results in dwarfism. Oversecretion of growth hormone results in giantism. Sex hormones, such as estradiol, are produced in higher amounts during and after puberty and can cause more rapid maturation and fusion of the epiphyseal plates. These hormones may limit the growth spurts of puberty, and early sexual maturity, especially in girls, can lead to shorter stature.
Bone Remodeling
Even in adulthood, bone is in a continuous state of turnover, a process that begins very early in skeletal development. This occurs in cycles of bone resorption and new bone formation called remodeling, with each complete cycle termed a bone remodeling unit. In a normal state, bone formation and resorption are closely coupled and balanced, serving to maintain the skeleton’s peak strength by removal and repair of damaged areas, which contributes to osteocyte viability and impacts the body’s calcium homeostasis.2
A bone remodeling unit occurs in a sequence of phases: stimulation and recruitment of osteoclasts to the area, resorption of bone, reversal and stimulation of osteoblasts, secretion of new matrix, mineralization of new bone, and completion of a resting phase.4 The stimulation of a remodeling cycle is thought to occur with osteoblastic production of factors that stimulate the recruitment and activity of osteoclasts (such as osteoclast differentiating factor, also called receptor activator of nuclear factor κB ligand, or RANKL). One such signal of the need for bone remodeling could come from mature osteocytes within the bone sensing bone deformation or damage.2 Osteoclasts gather at the site on the bone surface and form large multinucleated cells that begin the process of removal of bone matrix and minerals. This resorptive phase creates a pit in the bone, which is next filled with osteoblasts that begin to fill the space with bone matrix (osteoid). When the bone pit is filled, the new bone matrix is mineralized to complete the cycle (Figure 50-5).
Calcium Homeostasis
Almost all of the body’s calcium supply is held within bone, but the small amount of calcium circulating in blood is essential for a wide variety of cellular functions and therefore its concentration is tightly controlled. Much of this regulation of calcium balance in bone and blood depends on hormonal effects. Parathyroid hormone maintains serum calcium levels by increasing bone resorption as well as calcium reabsorption from renal tubules. Vitamin D metabolites can increase bone mineralization by increasing calcium absorption from the intestinal tract; however, in the setting of calcium deficiency, vitamin D can stimulate bone resorption to help maintain mineral supply in the blood. Calcitonin can act as an inhibitor of bone resorption but likely only plays a minor role in adults. (See Chapter 51 for further discussion.4)
Response to Injury, Stress, and Aging
The ability of bone to remodel after injury is important. Although remodeling of bone continues throughout life, death of the osteon or removal of calcium from bone requires that new bone be deposited to retain strength and function. Physical stresses lead to the realignment of bone trabecular systems and the deposition of additional bone at the site of increased stress. The response of bone to stress is summarized by Wolff’s law, which states that bone is laid down where it is needed and resorbed where it is not needed.5 If bone is immobilized or not subjected to mechanical stress, as occurs with prolonged bed rest, the activity of bone-resorbing cells increases.6 Without external forces (or loads), osteoclast activity is greater than osteoblast activity and bone mass decreases. It is probable that during bed rest, age-related bone loss might be temporarily accelerated and may result in a greater decline in bone mass over time. Patients becoming mobile after prolonged bed rest are at risk for fractures because of a combined loss of muscle and bone strength. With loss of muscle, gait becomes unsteady and patients are more prone to falls.
Internal fixation of a fracture may also cause decreased bone strength. With metal implants, mechanical stress is dispersed from bone and carried by the implant. Bone under the plate is resorbed, and “stress relief” osteoporosis may occur. Care must be taken once implants are removed, and the bone must be protected until strength returns. Some implants are designed to compress fracture fragments to aid healing.
Bone mass decreases with age. Studies have shown that elderly individuals express higher levels of certain markers associated with bone resorption, whereas bone formation markers are much more variable. One common cause of increased bone resorption is calcium and vitamin D deficiency, which causes more rapid mobilization of calcium from bone. A secondary hyperparathyroidism can also result. Decreased levels of estrogen in elderly women and men can contribute to age-related bone loss because osteoblasts have estrogen receptors and their ability to increase bone formation may be affected by the estrogen deficiency. An increase in the local production of cytokines that influence bone resorption may also occur with decreased estrogen levels. The end result is an imbalance between osteoblast and osteoclast function and progressive decline in bone mass4 (see Geriatric Considerations: Changes in the Skeletal System).
Bone mass can also decrease with certain disease processes. For example, osteoporosis is a metabolic bone disease characterized by a severe general reduction in skeletal bone mass and thus a susceptibility to fractures. In short, bone resorption is more rapid than bone formation.
Fracture Healing
Bone may heal in one of two ways after a fracture. A periosteal or external callus forms in fractures managed by closed methods. The blood supply to surrounding soft tissue and motion at the fracture site contribute to healing. Medullary callous formation takes place with rigid immobilization at the fracture site. The process of bone turnover contributes to healing.
The five stages of fracture healing are: (1) hematoma formation, 1 to 3 days; (2) fibrocartilage formation, 3 days to 2 weeks; (3) callous formation, 2 to 6 weeks; (4) ossification, 3 weeks to 6 months; and (5) consolidation/remodeling, 6 weeks to 1 year (Figure 50-6). These five stages can be grouped into three phases: (1) inflammatory phase, (2) reparative phase (stages 2 to 4), and (3) remodeling phase.
Stage 1 begins when a hematoma forms at the fracture site. The size of the hematoma depends on the amount of damage at the fracture site. The hematoma offers some stability to fractured ends. Aseptic inflammation occurs at the fracture site.
Healing continues during stage 2 with the formation of granular tissue containing blood vessels, fibroblasts, and osteoblasts. The hematoma provides the foundation for reparative tissue and bone healing. Vascular and mechanical factors such as motion and distraction of fragments influence stage 2.
Callous formation occurs during stage 3 after the granulation tissue matures. If this stage is delayed or interrupted, the final stages cannot occur.
Stage 4, or ossification, occurs as the space in the bone is bridged and the fractured ends are united. The callus is slowly replaced by trabecular bone along the lines of stress, and unnecessary callus is reabsorbed.
During stage 5, consolidation and remodeling occur as the medullary canal is reestablished. Bone is resorbed and deposited along stress lines as bone reshapes to meet its mechanical requirements.
Fractures are usually considered healed when clinical healing is achieved. Clinical healing occurs when the fracture is stable and strong enough to resume its function, the fracture site is free of pain, no gross movement is seen across the fracture site, and radiographs show bone crossing the fracture site.
Structure and Function of Joints
Coordinated movement is only possible because of joint, bone, and muscle structure. Joints permit complex, highly coordinated, and purposeful movements. A joint, also called an articulation, is a point of contact between bones. Functional articulations between bones in extremities such as the shoulder, elbow, hip, and knee contribute to controlled and graceful movement.
The type and configuration of a joint depend on the functional demands placed on that joint. As is the case with all aspects of the musculoskeletal system, structure determines function (Figure 50-7). When considering the human joint, or articulation, it is also important to remember that once the articulation has developed, the configuration of the joint surface will determine the movement of the joint. Any aberrant joint movement has the potential to disrupt function and cause a breakdown in joint integrity.
Articulations can provide more than a single function, such as flexion and extension. Flexion, extension, adduction, abduction, rotation, opposition, and circumduction may all be functional movements of a joint. The more complex the movements, the more complex is the joint structure.
Broadly speaking, articulations, or arthroses, in the human body may be divided into two categories based on the composition of the joint and the method in which the joints unite the body components. The two categories are synarthroses, or fibrous and cartilaginous (nonsynovial) joints, and diarthroses, or synovial joints.
Synarthroses
Synarthroses have two subdivisions based on the type of connective tissue used to form the joint. Fibrous and cartilaginous tissues give these joints their names.
Fibrous Structure
In a fibrous joint, bones are united by fibrous tissue. Three types of fibrous joints are found in the human body: suture joints, gomphosis joints, and syndesmosis joints. A suture joint unites bones with a thin but dense layer of fibrous tissue. Interlocking bony ends overlap and increase stability. Suture joints are found only in the skull (Figure 50-8). Fusion of the joint occurs later in life. This bony union is called a synostosis.1
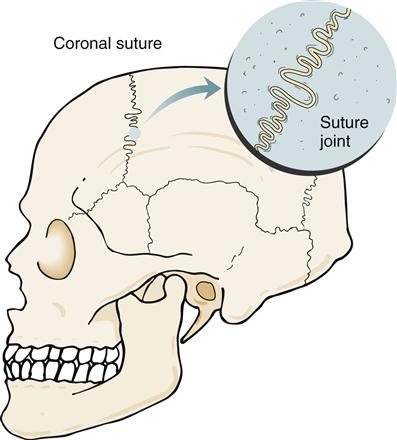
The joint that is found between a tooth and the mandible or maxilla is the only gomphosis joint in the human body. The best description of a gomphosis joint is that of a peg implanted into a hole. Fibrous tissue stabilizes the two bony structures and permits little movement.
A syndesmosis joint is a joint in which the two bony components are joined by a ligament or interosseous membrane. These joints normally allow slight movement and are quite functional. The interosseous membrane joining the fibula and the tibia is an example of a syndesmosis joint (Figure 50-9).
Cartilaginous Structure
Bony segments connected by fibrocartilage or hyaline growth cartilage are classified as cartilaginous joints. Symphysis joints and synchondrosis joints are the two types of cartilaginous joints in the body.
A symphysis joint connects bony segments by a fibrocartilaginous plate or disk. The symphysis pubis joint (Figure 50-10) joins the two pubic bones of the pelvis. This joint is a weight-bearing structure and is important in transmitting stress and providing stability. Little or no motion is permitted or desired.
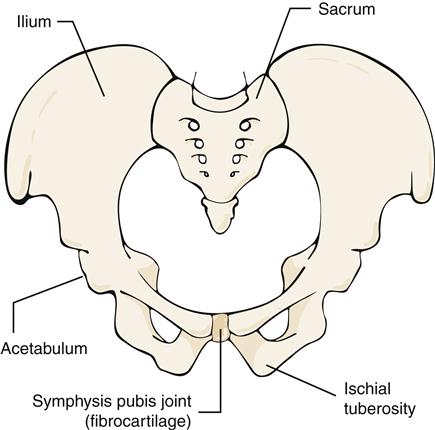
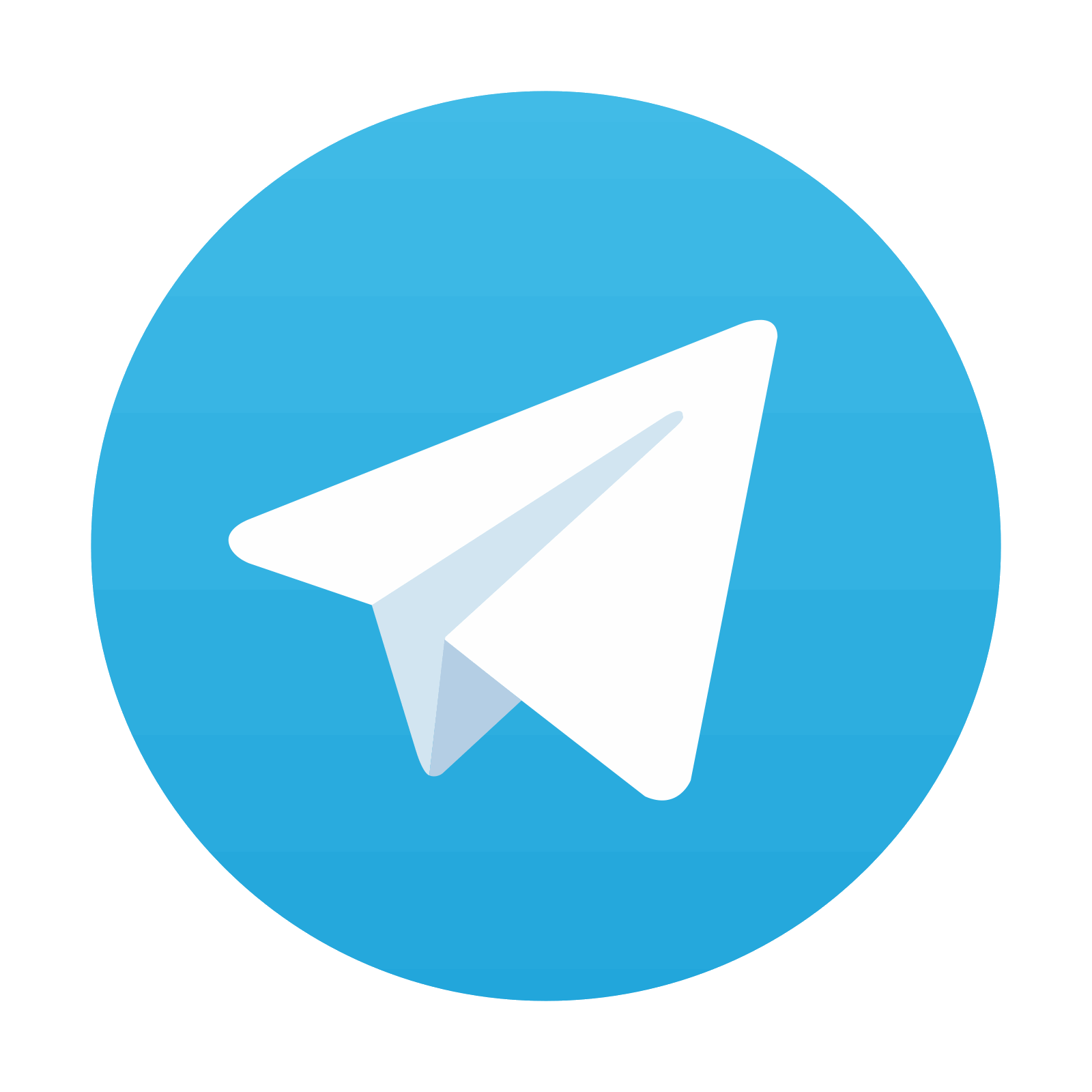
Stay updated, free articles. Join our Telegram channel
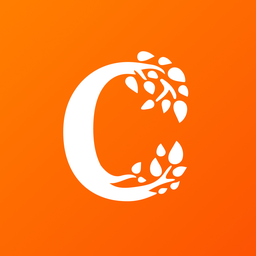
Full access? Get Clinical Tree
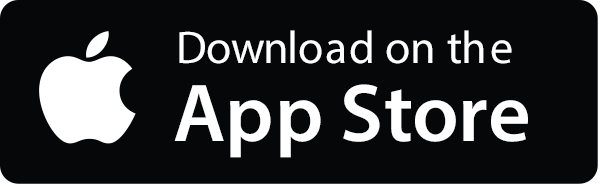
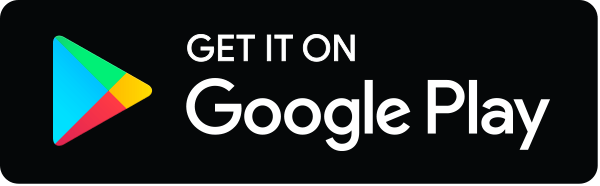