Chapter 31
Structure and Function of the Cardiovascular and Lymphatic Systems
Susanna G. Cunningham, Valentina L. Brashers and Kathryn L. McCance
A critical component of the circulatory system is the vascular endothelium, which is considered by some to be a separate organ. It is a multifunctional tissue whose health is essential to normal vascular and hemostatic physiology and whose dysfunction is an important factor in the pathogenesis of vascular and other diseases.1
Circulatory System
The heart is comprised of two conjoined pumps that power the movement of blood through two separate circulatory systems, one to the lungs and one to all other parts of the body. Structures on the right side of the heart, or right heart, pump blood through the lungs. (This system, termed the pulmonary circulation, is described in Chapter 34.) The left side of the heart, or left heart, sends blood throughout the systemic circulation, which supplies all of the body except the lungs (Figure 31-1). These two systems are serially connected so that the output of one pump becomes the input of the other.
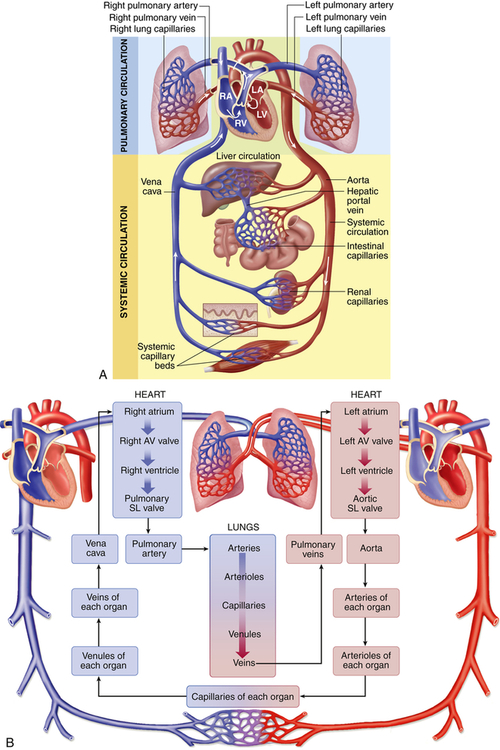
A, Right heart chambers propel unoxygenated blood through the pulmonary circulation, and the left heart propels oxygenated blood through the systemic circulation. B, The direction of blood flow begins at the left ventricle of the heart; flows to the arteries, arterioles, capillaries of each body organ, venules, veins, right atrium, right ventricle, pulmonary artery, lung capillaries, pulmonary veins, and left atrium; and then returns to the left ventricle. LA, Left atrium, LV, left ventricle; RA, right atrium; RV, right ventricle; SL, semilunar. (A from Patton KT, Thibodeau GA: Anatomy & physiology, ed 8, St Louis, 2013, Mosby. B from Thibodeau GA, Patton KT: The human body in health & disease, ed 6, St Louis, 2014, Mosby.)
Arteries carry blood from the heart to all parts of the body, where they branch into arterioles and even smaller vessels until they become a fine meshwork of capillaries. Capillaries allow the closest contact and exchange between the blood and the interstitial space, or interstitium—the environment in which the cells live. Veins then carry blood from capillaries back to the heart. Some of the plasma or liquid component of the blood passes through the walls of the capillaries into the interstitial space. This fluid, called lymph, is returned to the cardiovascular system by vessels of the lymphatic system. The lymphatic system is also a critical component of the immune system as described in Chapter 8.
The Heart
The adult heart weighs between 200 and 350 g and is about the size of a fist. It lies obliquely (diagonally) in the mediastinum, the area above the diaphragm and between the lungs. Women’s hearts are typically about 0.40% of their total body weight whereas men’s hearts are about 0.45% of total body weight.2
Heart structures can be categorized by function:
1. Structural support of heart tissues and circulation of pulmonary and systemic blood through the heart. This category includes the heart wall and fibrous skeleton, which enclose and support the heart and divide it into four chambers; the valves that direct flow through the chambers; and the great vessels that conduct blood to and from the heart.
2. Maintenance of heart cells. This category comprises vessels of the coronary circulation—the arteries and veins that serve the metabolic needs of all the heart cells—and the lymphatic vessels of the heart.
3. Stimulation and control of heart action. Among these structures are the nerves and specialized muscle cells that direct the rhythmic contraction and relaxation of the heart muscles, propelling blood throughout the pulmonary and systemic circulatory systems.
Structures That Direct Circulation Through the Heart
Heart Wall
The heart wall has three layers—the epicardium, myocardium, and endocardium—and is enclosed in a double-walled membranous sac, the pericardium (Figure 31-2). The pericardial sac has several functions. It (1) prevents displacement of the heart during gravitational acceleration or deceleration, (2) acts as a physical barrier that protects the heart against infection and inflammation from the lungs and pleural space, and (3) contains pain receptors and mechanoreceptors that can elicit reflex changes in blood pressure and heart rate. The outer layer of the pericardium, the parietal pericardium, is composed of a surface layer of mesothelium over a thin layer of connective tissue. The visceral pericardium, or epicardium, is the inner layer of the pericardium. At one point the visceral pericardium folds back and becomes continuous with the parietal pericardium, allowing the large vessels to enter and leave the heart without breaching the pericardial layers.
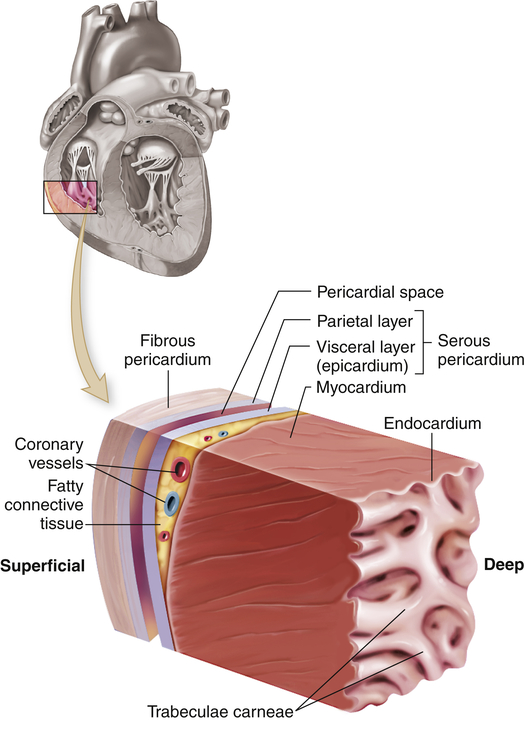
The cutout section of the heart wall shows the outer fibrous pericardium and the parietal and visceral layers of the serous pericardium (with the pericardial space between them). Note that a layer of fatty connective tissue is located between the visceral layer of the serous pericardium (epicardium) and the myocardium. Note also that the endocardium covers beamlike projections of myocardial muscle tissue, called trabeculae carneae. (From Patton KT, Thibodeau GA: Anatomy & physiology, ed 8, St Louis, 2013, Mosby.)
The visceral and parietal pericardia are separated by a fluid-containing space called the pericardial cavity. The pericardial fluid (about 20 ml), which is secreted by cells of the mesothelium, lubricates the membranes that line the pericardial cavity, enabling them to slide over one another with a minimum of friction as the heart beats.2 The amount and character of the pericardial fluid are altered by inflammation of the pericardium (see Chapter 32).
The outer layer of the heart, the epicardium, provides a smooth surface that allows the heart to contract and relax within the pericardium with a minimal amount of friction. The thickest layer of the heart wall, the myocardium, is composed of cardiac muscle and is anchored to the heart’s fibrous skeleton. The thickness of the myocardium varies tremendously from one heart chamber to another. Thickness is related to the amount of resistance the muscle must overcome to pump blood from the different chambers. The internal lining of the myocardium is composed of connective tissue and a layer of squamous cells called the endocardium (see Figure 31-2). The endocardial lining of the heart is continuous with the endothelium that lines all the arteries, veins, and capillaries of the body, creating a continuous, closed circulatory system (see What’s New? The Potential for Using Epicardial Cells in Myocardial Repair).
Chambers of the Heart
The heart has four chambers: the right atrium, left atrium, right ventricle, and left ventricle. These chambers form two pumps in series: the right heart, which is a low-pressure system pumping blood through the lungs; and the left heart, which is a high-pressure system pumping blood through the rest of the body. (Blood flow through these chambers is illustrated in Figure 31-3.) The atria are smaller than the ventricles and have
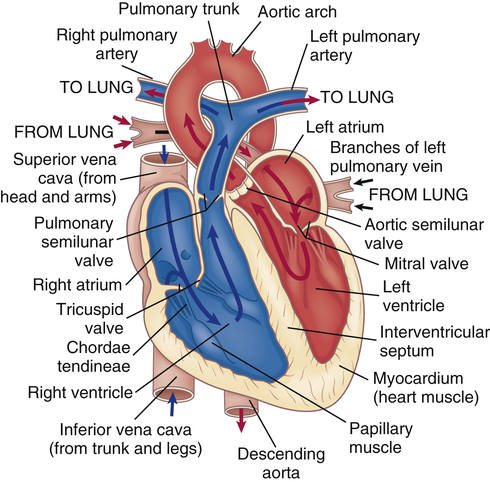
Arrows indicate path of blood flow through chambers, valves, and major vessels.
thinner walls. The walls of the right and left atria are about 1 to 2 mm thick. The ventricles have a thicker myocardial layer and make up much of the bulk of the heart. The wall of the right ventricle is about 4 to 5 mm thick, and that of the left ventricle, the most muscular chamber, is about 12 to 15 mm.2 The ventricles are formed by a continuum of muscle fibers that originate from the fibrous skeleton at the base of the heart (chiefly around the aortic orifice).
The ventricles are structurally more complex than the atria. Each ventricle contains muscle fibers that divide it roughly into an inflow tract, which receives blood from the atrium, and an outflow tract, which sends blood to the circulation (see Figure 31-3).
Normally blood does not flow between the chambers of the right side of the heart and the chambers of the left side of the heart except in the fetus before delivery. The adult right and left sides of the heart are separated by intact septal membranes. The atria are separated by the interatrial septum and the ventricles by the interventricular septum. There is an opening between the right and left atria before birth called the foramen ovale; however, this opening closes shortly after birth in most individuals (see Chapter 33). The interventricular septum is an extension of the fibrous skeleton of the heart. Indentations of the endocardium form valves that separate the atria from the ventricles and the ventricles from the aorta and pulmonary arteries.
Valves of the Heart
One-way blood flow through the heart is ensured by the four heart valves as well as the pressure gradients that they maintain. During ventricular relaxation the two atrioventricular valves open and blood flows from the relatively higher pressure in the atria to the lower pressure in the relaxed ventricles. With increasing ventricular pressure these valves close and prevent backflow into the atria as the ventricles contract. The semilunar valves of the heart open when intraventricular pressure exceeds aortic and pulmonary pressures and blood flows out of the ventricles and into the systemic and pulmonary circulations, respectively. After ventricular contraction and ejection, intraventricular pressure falls and the pulmonic and aortic semilunar valves close when the pressure in the vessels is greater than the pressure in the ventricles, thus preventing backflow into the right and left ventricles, respectively (Figure 31-4; also see Figure 31-3).
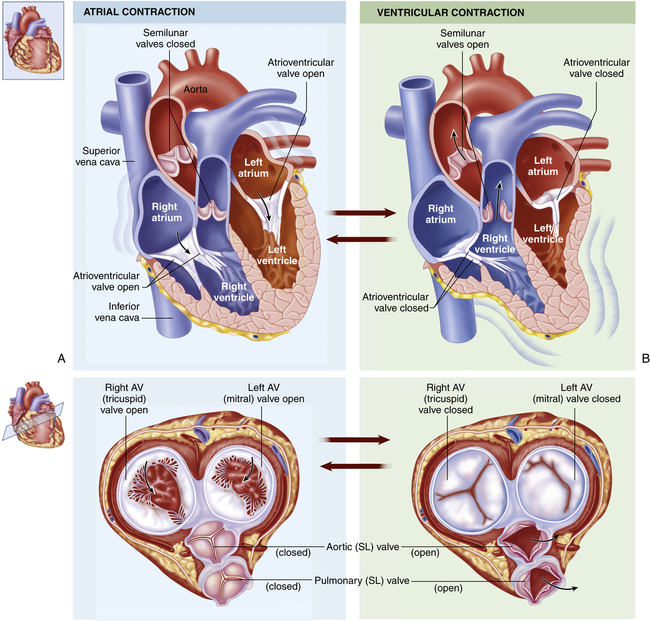
Chambers and valves of the heart. A, During atrial contraction cardiac muscle in the atrial wall contracts, forcing blood through the atrioventricular (AV) valves and into the ventricles. Bottom illustration shows superior view of all four valves, with semilunar (SL) valves closed and AV valves open. B, During ventricular contraction that follows, the AV valves close and the blood is forced out of the ventricles through the SL valves and into the arteries. Bottom illustration shows superior view of SL valves open and AV valves closed. (From Patton KT, Thibodeau GA: Anatomy & physiology, ed 8, St Louis, 2013, Mosby.)
The atrioventricular (AV) (tricuspid and mitral) valve openings are composed of flaps of tissue called leaflets or cusps that are attached at the upper end to one of the rings in the fibrous skeleton of the heart and at the lower end to papillary muscles by the chordae tendineae (see Figure 31-3). The papillary muscles are extensions of the myocardium that help hold the cusps together and downward at the onset of ventricular contraction, thus preventing their backward expulsion, or prolapse, into the atria (see p. 1087 for a description of pressure changes and valvular function).
Blood leaves the right ventricle through the pulmonic semilunar valve, and it leaves the left ventricle through the aortic semilunar valve (see Figures 31-3 and 31-4). The pulmonic and aortic semilunar valves have three cup-shaped cusps that arise from the fibrous skeleton. The pulmonic cusps are slightly thinner than the aortic cusps. The lower edges of each cusp are suspended from the root of the pulmonary artery or aorta, with the upper valve edges freely projecting into the vessel lumen. When the ventricles contract, the cusps behave like one-way swinging doors. The force of the blood propels the cusps outward against the vessel wall. When the ventricles relax, blood fills the cusps and causes their free edges to meet in the middle of the vessel, closing the valve and preventing any backflow.
Great Vessels
Blood moves in and out of the heart through several large vessels (see Figure 31-3). The right heart receives venous deoxygenated blood from the systemic circulation through the superior vena cava and the inferior vena cava, which enter the right atrium. Blood leaves the right ventricle and enters the pulmonary circulation through the pulmonary artery. The pulmonary artery divides into right and left pulmonary arteries to transport unoxygenated blood from the right heart to the right and left lungs. The pulmonary arteries branch further into the pulmonary capillary bed, where oxygen enters the blood and carbon dioxide leaves it as each gas moves from its higher to lower concentration gradient.
Blood Flow During the Cardiac Cycle
The pumping action of the heart consists of contraction and relaxation of the heart muscle or myocardium. Each ventricular contraction and the relaxation that follows it constitute one cardiac cycle. (Blood flow through the heart during a single cardiac cycle is illustrated in Figure 31-4.) During the period of relaxation, termed diastole, blood fills the ventricles. The contraction that follows, termed systole, propels the blood out of the ventricles and into the pulmonary and systemic circulations. Contraction of the left ventricle occurs slightly earlier than contraction of the right ventricle.
During ventricular systole, blood from the veins of the systemic circulation enters the thin-walled right atrium from the superior and inferior venae cavae (see Figures 31-3 and 31-4). Venous blood from the coronary circulation enters the right atrium through the coronary sinus. The right atrium fills, which, along with the falling right ventricular pressures, allows the right AV (tricuspid) valve to open and fill the right ventricle during ventricular diastole (occasionally called atrial systole). The same sequence of events occurs a split second earlier in the left heart. The four pulmonary veins, two from the right lung and two from the left lung, carry blood from the pulmonary circulation to the left atrium. As the left atrium fills and left ventricular pressure falls, the mitral valve opens and blood flows into the left ventricle. Left atrial contraction, termed “atrial kick,” provides significant increases in the volume of blood entering the left ventricle at the end of diastole. Filling of the right and left ventricles occurs during one period of diastole.
Five phases of the cardiac cycle can be identified (Figures 31-5 and 31-6):
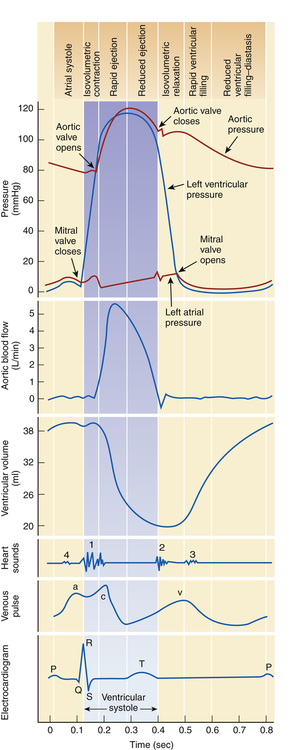
This chart is a composite of several diagrams of heart function (blood pressure, blood flow, volume, heart sounds, venous pulse, and electrocardiogram [ECG]), all adjusted to the same time scale.
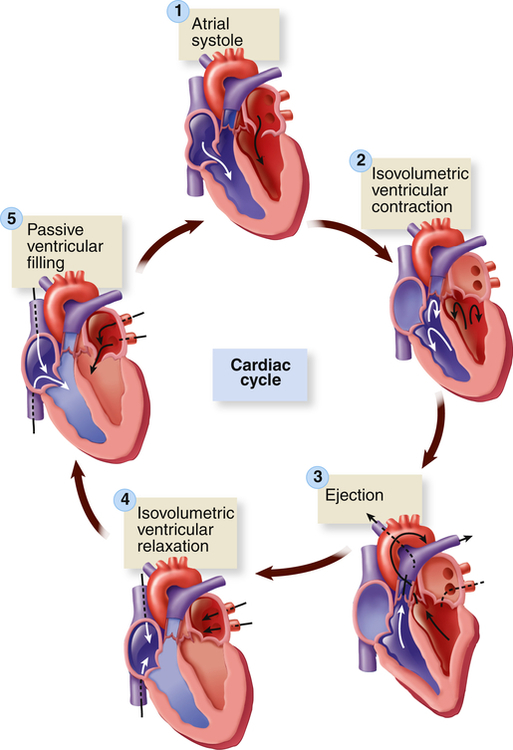
1, Atrial systole. 2, Isovolumetric ventricular contraction. Ventricular volume remains constant as pressure increases rapidly. 3, Ejection. 4, Isovolumetric ventricular relaxation. Both sets of valves are closed, and the ventricles are relaxing. 5, Passive ventricular filling. The atrioventricular (AV) valves are forced open, and the blood rushes into the relaxing ventricles. (From Patton KT, Thibodeau GA: Anatomy & physiology, ed 8, St Louis, 2013, Mosby.)
Phase 1: Ventricular diastole or atrial systole begins with opening of the mitral and tricuspid valves and then ventricular filling from the atria occurs. The ventricles fill rapidly in early diastole and again in late diastole when the atria contract.
Phase 2: Ventricular systole begins with “isovolumetric contraction,” so-called because ventricular volume is constant since both the AV and the semilunar valves are closed. The first detectable rise in ventricular pressure occurs during isovolumetric contraction. This contraction pushes the AV valves shut. Their cusps bulge slightly into the atria but are prevented from opening back into the atria by their anchors, the chordae tendineae (see Figure 31-3).
Phase 3: When ventricular pressure reaches and then slightly exceeds that of the pulmonary artery and aorta, the semilunar valves open and ventricular ejection occurs. Intraventricular pressure and ventricular volume decrease rapidly.
Phase 4: With ventricular relaxation and decreased ventricular pressure, the aortic valve closes and “isovolumetric relaxation” occurs. Again, both the AV and the semilunar valves are closed during this phase.
Phase 5: When left ventricular pressure falls below atrial pressure, the mitral and tricuspid valves open and passive ventricular filling occurs.
Normal Intracardiac Pressures
Normal intracardiac pressures are shown in Table 31-1 and Figure 31-7. Atrial pressure (see venous pulse in Figure 31-5) curves are composed of the a wave, which is generated by atrial contraction, and the v wave, which is an early diastolic peak caused by filling of the atrium from the peripheral veins. A smaller pressure increase, the c wave, occurs after the a wave in early systole and may represent bulging of the mitral valve into the left atrium during early systole. Two aspects of falling atrial pressure have also been named. The x descent follows the a wave and is produced by the descent of the tricuspid valve ring and by the ejection of blood from both ventricles. The y descent that follows the v wave reflects the rapid flow of blood from the great veins and right atrium into the right ventricle. Left ventricular pressures are illustrated by a peak systolic pressure and an end-diastolic pressure, which is the ventricular pressure immediately before the onset of systole. The minimal left ventricular pressure occurs in early diastole.
TABLE 31-1
MEAN (mmHg) | RANGE (mmHg) | |
Right atrium | 4 | 0-8 |
Right ventricle | ||
24 | 15-28 | |
4 | 0-8 | |
Left atrium | 7 | 4-12 |
Left ventricle | ||
130 | 90-140 | |
7 | 4-12 |
Structures That Support Cardiac Metabolism: The Coronary Vessels
To supply oxygen and other nutrients to the myocardium, heart structures are nourished by vessels of the systemic circulation, the coronary circulation. The coronary arteries receive blood through openings in the aorta, called the coronary ostia. The cardiac veins empty into the right atrium through another ostium, the opening of a large vein called the coronary sinus (Figure 31-8).
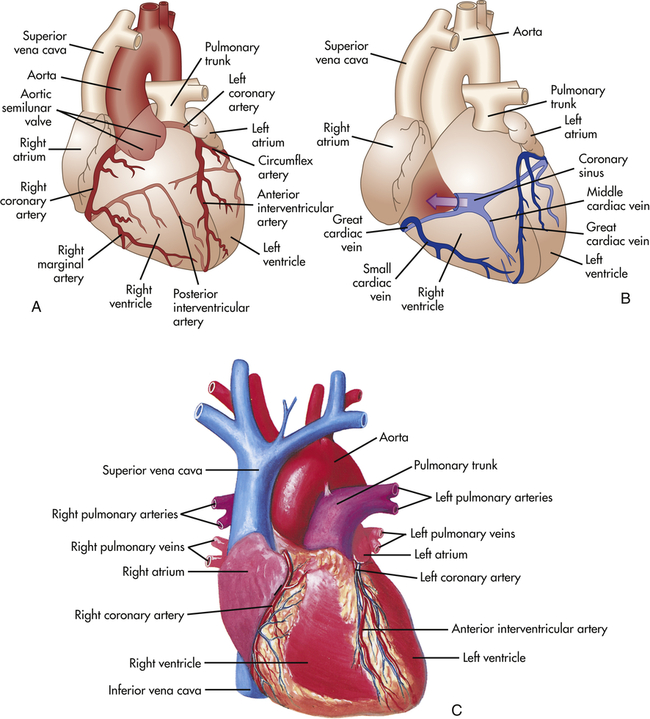
A, Arteries. B, Veins. Both A and B are anterior views of the heart. Vessels near the anterior surface are more darkly colored than vessels of the posterior surface seen through the heart. C, View of the anterior (sternocostal) surface. (A and B modified from Patton KT, Thibodeau GA: Anatomy & physiology, ed 8, St Louis, 2013, Mosby. C from Seeley RR, Stephens TD, Tate P: Anatomy & physiology, ed 3, St Louis, 1995, Mosby.)
Coronary Arteries
The major coronary arteries are the right coronary artery (RCA) and the left coronary artery (LCA) (see Figure 31-8). These arteries traverse the epicardium and branch several times. The right coronary artery has greater flow than the left in 70% of individuals, the left greater than the right in 10%, and equal flow in each is found in 20% of individuals.3 The pattern of branching through the visceral pericardium differs among individuals. The branches of the coronary arteries enter the myocardium and endocardium and branch further to become arterioles and then capillaries. The coronary arteries are smaller in women than in men, a fact that is attributed to differences in heart weight.
The circumflex artery travels in a groove called the coronary sulcus, which separates the left atrium from the left ventricle, to the left border of the heart. It supplies blood to the left atrium and the lateral wall of the left ventricle. The circumflex artery often branches to the posterior surfaces of the left atrium and left ventricle (see Figure 31-8).
Collateral Arteries
The functional importance of the collateral circulation is that it protects the heart from ischemia. The collateral circulation is responsible for supplying blood and oxygen to the myocardium that has been deprived of oxygen following narrowing of a major coronary artery (coronary artery disease). Gradual coronary occlusion results in the growth of coronary collaterals. New collateral vessels are formed through two processes: arteriogenesis (new artery growth branching from pre-existing arteries) and angiogenesis (growth of new capillaries within a tissue).4 A key stimulus to collateral growth is the shear stress caused by increased blood flow velocity that occurs close to sites of stenosis (narrowing) or occlusion. Shear stress activates the endothelium of the pre-existing arterioles and stimulates the production of growth factors and cytokines, including monocyte chemoattractant protein-1 (MCP-1) and vascular endothelial growth factor (VEGF).5 Current research is focused on identifying whether some of these factors that stimulate collateral growth might prove to be useful treatments for persons with myocardial ischemia, although to date none have been demonstrated to be effective.5
Coronary Capillaries
Alterations of the cardiac muscles dramatically affect blood flow in the capillaries. For example, in ventricular hypertrophy (enlargement of the ventricular myocardium), the capillary network does not expand along with muscle fiber size. Therefore, the same number of capillaries must now perfuse a larger area. This results in decreased exchange of oxygen and nutrients. At rest, the heart extracts 70% to 80% of the oxygen delivered to it and coronary blood flow is directly correlated with myocardial oxygen consumption.6
Coronary Veins and Lymphatic Vessels
After passing through the extensive capillary network, blood from the coronary arteries drains into the cardiac veins, which travel alongside the arteries. Most of the venous drainage of the heart occurs through veins in the visceral pericardium. The veins then feed into the great cardiac vein (see Figure 31-8) and coronary sinus on the posterior surface of the heart, between the atria and ventricles, in the coronary sulcus. Venous coronary blood empties into the right atrium from the coronary sinus. Blood from the left ventricular walls generally is drained through the coronary sinus and its tributaries, which together form the largest system of coronary veins. The anterior interventricular vein flows beside the LAD artery and then turns to join the circumflex artery and becomes the great cardiac vein that primarily drains the anterior surface of the heart. The posterior vein of the left ventricle, the largest on the posterior surface of the heart, branches from the coronary sinus and accompanies the circumflex artery and then joins the great cardiac vein.3
The myocardium has an extensive system of lymphatic capillaries and collecting vessels within the layers of the myocardium and also in the valves. With cardiac contraction the lymphatic vessels drain fluid to the paratracheal lymph nodes in the anterior mediastinum and then continue to join the mediastinal lymphatic vessels. Impairment of cardiac lymphatic function has been hypothesized to impact cardiac conduction, protection from infection, and the development of myocardial fibrosis and atherosclerosis.7
Structures That Control Heart Action
Our lives depend on the continuous repetition of the cardiac cycle (systole and diastole) that occurs because of the transmission of electrical impulses, termed cardiac action potentials, through the myocardium. (Action potentials are described in Chapters 1 and 3.) As an electrical impulse passes from cell to cell (fiber to fiber) in the myocardium, it stimulates an intracellular process that results in fiber shortening—that is, muscular contraction or systole. Between action potentials, the fibers relax and return to their resting length, causing diastole. The muscle fibers of the myocardium are electrically coupled so that action potentials pass from cell to cell very rapidly and efficiently. The myocardial structures that allow the action potentials to move so rapidly through the heart are the gap junctions in the intercalated disks. In the intercalated disks, the channel-forming proteins, called connexins, form pores in the gap junctions.8 As a result of these structures plus the heart’s conduction system, an action potential generated in one part of the myocardium passes very quickly throughout the heart, causing rapid, organized, sequential contraction of the atria and then the ventricles.
The myocardium differs from skeletal muscle tissue in that it contains its own pacemakers and conduction system—specialized cells that enable it to generate and transmit action potentials without input from the nervous system (Figure 31-9). The pacemaker cells are concentrated at two sites in the myocardium called nodes, the sinoatrial (SA) node and the atrioventricular (AV) node. Although the heart is innervated by the autonomic nervous system (sympathetic and parasympathetic fibers), neural impulses are not needed to maintain the cardiac cycle. Thus the heart will beat in the absence of any nervous connection, one of the many factors that allow heart transplantation to be successful. The cardiac cycle is stimulated by the nodes of specialized cells and then adjusted to the physical needs of the body by the autonomic fibers. The sympathetic and parasympathetic nerves affect the speed of the cardiac cycle (heart rate, or beats per minute), the force of contraction, and the diameter of the coronary vessels (Figure 31-10). The sympathetic nervous system increases heart rate and conduction through the nodes, the parasympathetic nervous system slows heart rate and prolongs intranodal conduction time, and both systems cause coronary vasodilation.9
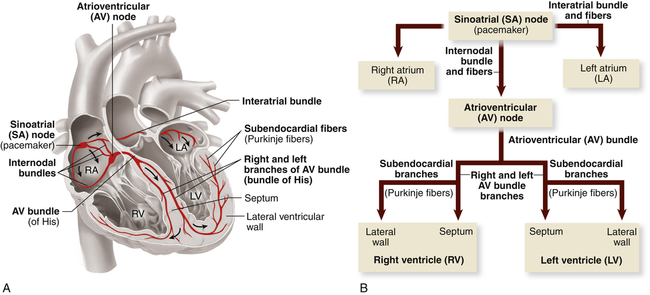
Specialized cardiac muscle cells (boldface type) in the wall of the heart rapidly initiate or conduct an electrical impulse throughout the myocardium. Both the sketch of the conduction system (A) and the flowchart (B) show the origin and path of conduction. The signal is initiated by the SA node (pacemaker) and spreads to the rest of the right atrial myocardium directly, to the left atrial myocardium by way of a bundle of interatrial conducting fibers, and to the AV node by way of three internodal bundles. The AV node then initiates a signal that is conducted through the ventricular myocardium by way of the AV bundle (of His) and subendocardial branches (Purkinje fibers). (From Patton KT, Thibodeau GA: Anatomy & physiology, ed 8, St Louis, 2013, Mosby.)
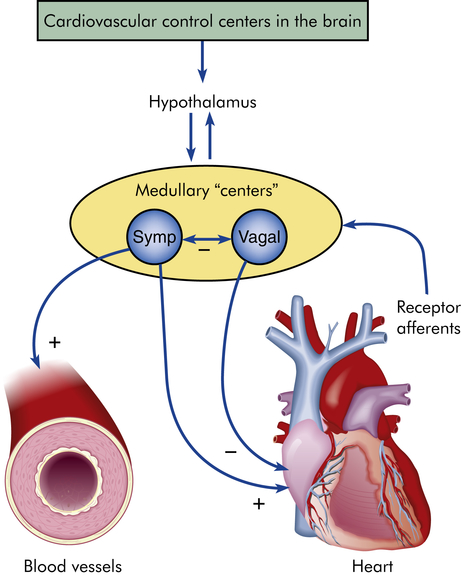
+, Activation; −, inhibition; Symp, sympathetic.
Conduction System
Normally electrical impulses arise in the sinoatrial (SA) node (SA node, sinus node), the usual pacemaker of the heart. The SA node is located at the junction of the right atrium and superior vena cava, just above the tricuspid valve (see Figure 31-9). The SA node sits only about 1 mm beneath the visceral pericardium, making it vulnerable to injury and disease, especially pericardial inflammation. The SA node is nourished by the sinus node artery, which passes through the center of the node. The SA node is heavily innervated by both sympathetic and parasympathetic nerve fibers. Impulse formation is thought to occur in two cell types found in the node—spindle- and spider-shaped cells.9
In the resting adult the SA node generates about 60 to 100 action potentials per minute depending on age and physical condition. Each action potential travels rapidly from cell to cell and through special pathways in the atrial myocardium, causing both atria to contract, beginning systole. The action potential is transmitted from the atrial to the ventricular myocardium through fibers of the conduction system, traveling first to the atrioventricular (AV) node, then to the bundle of His (atrioventricular bundle, common bundle), and finally through the bundle branches of the interventricular septum to Purkinje fibers in the heart wall (see Figure 31-9).
The AV node is well situated for mediating conduction between the atria and ventricles. It is located in the right atrial wall above the tricuspid valve and anterior to the ostium of the coronary sinus. There is variability in the size and length of the AV node fibers. Generally the AV node is thicker and shorter in size than the SA node. Behind the AV node are numerous autonomic parasympathetic ganglia. (The nervous systems are described in Chapter 15.)
Cardiac Excitation
From the SA node the impulse that begins systole spreads throughout the right atrium at a conduction velocity of about 35 cm/sec.9 Because impulses from the SA node arrive at the AV node very quickly, investigators have proposed that these nodes are connected by internodal pathways, called the anterior, middle, and posterior internodal pathways. However, the existence of these pathways is controversial; not all experts agree that they exist.9
The action potential is delayed in the region of the AV node, possibly because of electrophysiologic differences in the cells that comprise the AV region. Conduction velocity within the node is about 10 cm/sec, markedly slower than conduction through the atria.9 The delay between atrial and ventricular excitation permits an additional boost to ventricular filling by atrial contraction (atrial kick). From the AV node the impulse travels from the AV bundle and through the bundle branches to the Purkinje fibers. Conduction velocities in the AV and Purkinje fibers are the most rapid in the heart.
Ventricular activation occurs sequentially in three phases: (1) septal activation, (2) apical activation, and (3) basal (upper) and posterior activation. The first areas of the ventricles to be excited are portions of the interventricular septum. The septum is activated from both the RBB and the LBB, although the impulse travels from left to right. The extensive network of Purkinje fibers promotes the rapid spread of the impulse to the ventricular apexes. Activation traverses the heart wall from the inside outward (from the endocardium to the epicardium; see Figure 31-2). The basal and posterior portions of the ventricles are the last to be activated. Deactivation, which begins in diastole, occurs in the opposite direction, spreading from the outside inward (epicardium to endocardium). All areas of the ventricle recover at about the same time.
Propagation of Cardiac Action Potentials
Electrical activation of the muscle cells, termed depolarization, is caused by the movement of ions, including sodium, potassium, calcium, and chloride, across cardiac cell membranes. Deactivation, called repolarization, occurs the same way. (Movement of ions across cell membranes is described in Chapter 1; electrical activation of muscle cells is described in Chapter 43.)
Movement of ions into and out of the cell creates an electrical (voltage) difference across the cell membrane called the membrane potential. The resting membrane potential of myocardial cells is between −80 and −90 millivolts (mV), whereas the SA node is between −50 and −60 mV and the AV node is between −60 and −70 mV.9 During depolarization the inside of the cell becomes less negatively charged. In cardiac cells the difference between resting membrane potential (in millivolts) and the decreased negative charge caused by depolarization is the cardiac action potential. Table 31-2 summarizes the intracellular and extracellular ionic concentrations of cardiac muscle. The various phases of the cardiac action potential are related to changes in the permeability of the cell membrane to sodium, potassium, chloride, and calcium. Threshold is the point at which the cell membrane’s selective permeability to these ions is temporarily disrupted, leading to depolarization. If the resting membrane potential becomes more negative as a result of a decrease in extracellular potassium concentration (hypokalemia), it is termed hyperpolarization.
TABLE 31-2
INTRACELLULAR AND EXTRACELLULAR ION CONCENTRATIONS IN THE MYOCARDIUM
ION | INTRACELLULAR CONCENTRATION | EXTRACELLULAR CONCENTRATION |
Sodium (Na+) | 15 mM | 145 mM |
Potassium (K+) | 150 mM | 4 mM |
Chloride (Cl−) | 5-30 mM | 120 mM |
Calcium (Ca++) | 10−7 M | 2 mM |
M, Moles; mM, millimoles per kilogram.
From Bonow RO et al, editors: Braunwald’s heart disease: a textbook of cardiovascular medicine, ed 9, Philadelphia, 2012, Elsevier Saunders.
Normal myocardial cell depolarization and repolarization occur in five phases numbered 0 through 4 (Figure 31-11). Phase 0 consists of depolarization. This phase lasts 1 to 2 milliseconds (ms) and represents rapid sodium entry into the cell. Phase 1 is early repolarization, in which calcium slowly enters the cell. Phase 2, also called the plateau, is a continuation of repolarization, with slow entry of calcium and sodium into the cell. Potassium is moved out of the cell during phase 3, with a return to resting membrane potential in phase 4.9 The time between action potentials corresponds to diastole.
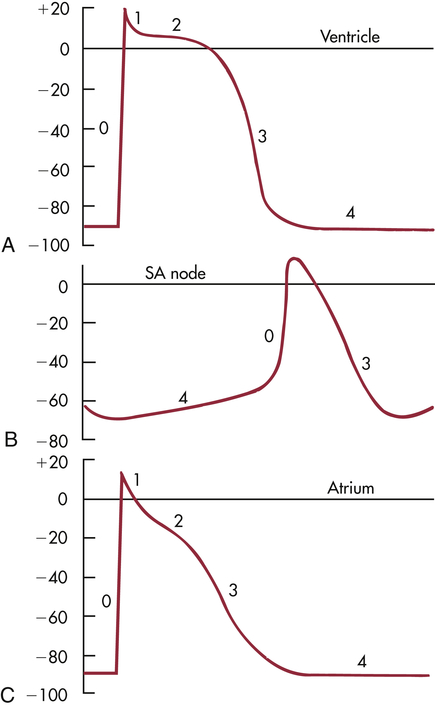
A, Ventricle. B, Sinoatrial (SA) node. C, Atrium. Sweep velocity in B is half that in A or C. (Modified from Koeppen BM, Stanton BA: Berne and Levy physiology, ed 6, Philadelphia, 2010, Mosby.)
The phases of depolarization and repolarization occur somewhat differently in the SA and AV node cells, a difference that enables these cells to generate cardiac action potentials independently. The cells of the Purkinje fibers, atria, and ventricles begin with a negative resting membrane potential and proceed to a rapid upstroke, or depolarization (phase 0), a rapid early repolarization (phase 1), a plateau (phase 2), and a rapid later repolarization (phase 3) (see Figure 31-11, A and C). The fast inward current of phase 0 is mediated by sodium ions flowing through “fast channels” in the cell membrane and causes the rapid upstroke of the action potential in Purkinje fibers, atria, and ventricles. In contrast, the cells of the SA and AV nodes begin with a less negative resting membrane potential, proceed to a slow upstroke (phase 0), and usually lack a plateau (phase 2) (see Figure 31-11, B). The slow inward current, mediated by calcium through transient and long-lasting channels and sodium ions flowing through “slow channels” of the cell membrane, is responsible for the action potential of the SA node and the AV node. Hence, drugs that block calcium have profound effects on the slow inward current and can alter heart rate. Slow channel-blocking drugs, such as verapamil, are used to treat a variety of cardiovascular disorders.
A refractory period, during which no new cardiac action potential can be initiated by a stimulus, follows depolarization. This effective or absolute refractory period corresponds to the time needed for the reopening of channels that permit sodium and calcium influx (phase 0 through half of phase 3). A relative refractory period occurs near the end of repolarization, following the effective refractory period. During this time the membrane can be depolarized again but only by a greater than normal stimulus. Abnormal refractory periods as a result of disease can cause abnormal heart rhythms, or dysrhythmias, including ventricular fibrillation and cardiac arrest (see Chapter 32).
Normal Electrocardiogram
The genesis of the normal electrocardiogram is from electrical activity recorded by skin electrodes, that is, the sum of all cardiac action potentials (Figure 31-12). The P wave represents atrial depolarization. The PR interval is a measure of time from the onset of atrial activation to the onset of ventricular activation; it normally ranges from 0.12 to 0.20 second. The PR interval represents the time necessary to travel from the sinus node through the atrium, AV node, and His-Purkinje system to activate ventricular myocardial cells. The QRS complex represents the sum of all ventricular muscle cell depolarizations. The configuration and amplitude of the QRS complex vary considerably among individuals. The duration is normally between 0.06 and 0.10 second. During the ST interval the entire ventricular myocardium is depolarized. The QT interval is sometimes called the “electrical systole” of the ventricles. It lasts about 0.4 second, but it varies inversely with the heart rate.
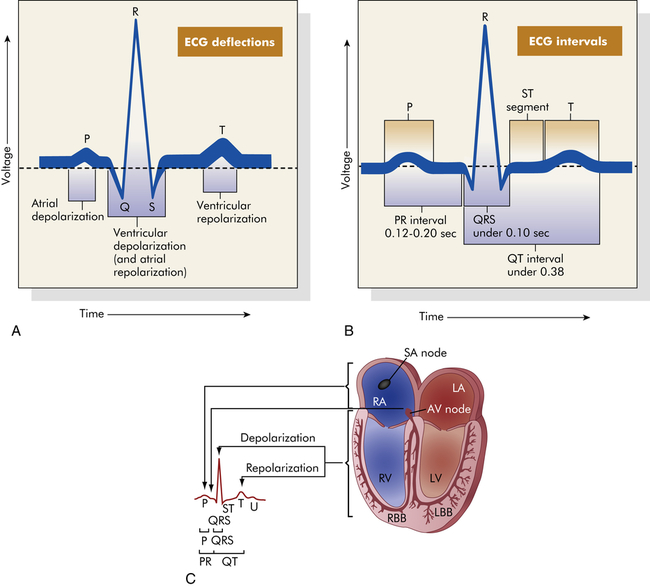
A, Normal ECG. Depolarization and repolarization. B, ECG intervals among P, QRS, and T waves. C, Schematic representation of ECG and its relationship to cardiac electrical activity. AV, Atrioventricular; LA, left atrium; LBB, left bundle branch; LV, left ventricle; RA, right atrium; RBB, right bundle branch; RV, right ventricle. (A and B from Thibodeau GA, Patton KT: Anatomy & physiology, ed 5, St Louis, 2003, Mosby. C from Thibodeau GA: Anatomy & physiology, St Louis, 1987, Mosby.)
Automaticity
Automaticity, or the property of generating spontaneous depolarization to threshold, enables the SA and AV nodes to generate cardiac action potentials without any stimulus. Cells capable of spontaneous depolarization are called automatic cells. The automatic cells of the cardiac conduction system can stimulate the heart to beat even when the heart is removed from the body. Spontaneous depolarization is possible in automatic cells because the membrane potential does not “rest” during phase 4. Instead, it slowly creeps toward threshold during the diastolic phase of the cardiac cycle. Because threshold is approached during diastole, phase 4 in automatic cells is called diastolic depolarization. The electrical impulse normally begins in the SA node because its cells depolarize more rapidly than other automatic cells. The ionic basis for this diastolic depolarization is the regular rhythmic oscillation of calcium with the automatic cells, a phenomenon known as the “calcium clock.”9
Cardiac Innervation
Although the heart’s nodes and conduction system generate cardiac action potentials independently, the autonomic nervous system influences the rate of impulse generation (firing), depolarization, and repolarization of the myocardium and the strength of atrial and ventricular contraction. Autonomic neural transmission produces changes in the heart and circulatory system faster than metabolic or humoral agents (see Figure 31-10). Speed is important, for example, in stimulating the heart to increase its pumping action during times of stress and fear, the so-called fight-or-flight response, or with increased physical activity. Although increased delivery of oxygen, glucose, hormones, and other blood-borne factors sustains increased cardiac activity, the rapid initiation of increased activity depends on the sympathetic and parasympathetic fibers of the autonomic nervous system. (The autonomic nervous system is described and illustrated in Chapter 15.)
Sympathetic and Parasympathetic Nerves
The efferent parasympathetic fibers originate in the medulla oblongata and travel by way of the vagus nerves to join the sympathetic nerves in the cardiac plexus. Parasympathetic (vagal) activity causes the release of acetylcholine. Receptors for these neurotransmitters are found in the myocardium and coronary vessels of the heart. Acetylcholine decreases heart rate, slows conduction through the AV nodes, and reduces myocardial contraction strength.9
Adrenergic Receptor Function
Sympathetic neural stimulation of the myocardium and coronary vessels depends on the presence of G-protein–coupled adrenergic receptors, which bind specifically with neurotransmitters of the sympathetic nervous system. (Receptor physiology is discussed in Chapter 1.) The effects of sympathetic stimulation depend on whether (1) the α- or β-adrenergic receptors are most plentiful on cells of the effector tissue, (2) the neurotransmitter is norepinephrine or epinephrine, and (3) the extent to which the individual variations in receptor structure caused by single nucleotide polymorphisms (SNPs) influence receptor responsiveness.10,11
There are five types of adrenergic receptors: β1, β2, β3, α1, and α2 (see Table 15-7). Each of the α-adrenergic receptors also has three subtypes, so some sources indicate that there are nine types of adrenergic receptors.11,12 Overall, cardiovascular structures have more β than α receptors; therefore, effects mediated by the β receptors predominate. Norepinephrine is released by postsynaptic sympathetic nerve endings in the heart while epinephrine is mainly released by the adrenal medulla and reaches the heart through the bloodstream.
The β1 receptors are found mostly in the heart, specifically the conduction system (AV and SA nodes, Purkinje fibers) and the atrial and ventricular myocardium. The β2 receptors are found in the heart and also on vascular smooth muscle. Stimulation of both the β1 and β2 receptors results in an increase in heart rate (chronotropy) and force of myocardial contraction (inotropy).13 In addition, stimulation of the β2 receptors results in vasodilation because of the location of the receptors on vascular smooth muscle. Overall β1 and β2 stimulation enables the heart to pump more blood and β2 stimulation also increases coronary blood flow, and β3 receptors are also found in the myocardium and coronary vessels. In the heart, stimulation of these receptors opposes the effects of β1– and β2-receptor stimulation and decreases myocardial contractility (negative inotropic effect).13 Thus β3 receptors may provide a “safety mechanism” to prevent overstimulation of the heart by the sympathetic nervous system.
Norepinephrine binding with α1 receptors, all of which are postsynaptic in the systemic and coronary arteries, causes smooth muscle contraction and thus vasoconstriction. One of the three subtypes of α2 receptors, α2a, is located on the sympathetic ganglia and nerve terminals. The effect of norepinephrine on these receptors is to inhibit release of more norepinephrine, which promotes vasodilation, thus providing another safety mechanism to prevent excess blood pressure elevation.13 Dysfunction of α- and β-adrenergic receptors can occur in many conditions (e.g., diabetes, hypertension) and has been implicated in the pathogenesis of many cardiac diseases, including heart failure, myocardial ischemia, and dysrhythmias.10,11,13
Myocardial Cells
The cells of cardiac muscle (the myocardium) and of skeletal muscle are nearly identical in structure, function, and microscopic appearance. (The properties of skeletal muscle are described in detail in Chapter 43.) Both types of muscle tissue are composed of long, narrow cells, called fibers, that contain basically the same structures: bundles of longitudinally arranged myofibrils; a single nucleus (cardiac muscle) or many nuclei (skeletal muscle); mitochondria; an internal membrane system (the sarcoplasmic reticulum); cytoplasm (sarcoplasm); and a plasma membrane (the sarcolemma), which encloses the cell. Cardiac and skeletal muscle cells also have an “external” membrane system made up of transverse tubules (T tubules) formed by invaginations of the sarcolemma. The sarcoplasmic reticulum forms a network of channels that surround the muscle fiber.
The microscopic appearance of cardiac and skeletal muscle is somewhat similar as well (see Chapter 1, Table 1-8). Because the myofibrils in both types of fibers consist of alternating light and dark bands of protein, the fibers appear striped, or striated. The dark and light bands of the myofibrils comprise longitudinal repeating units called sarcomeres. The length of the sarcomeres, normally between 1.6 and 2.2 mm, is important because it determines the limits of myocardial stretch at the end of diastole and subsequently the force of contraction during systole.
Rapid transmission of electrical impulses from cardiac fiber to cardiac fiber is possible because the network of fibers is connected at specialized intercellular junctions called intercalated disks. Intercalated disks are thickened portions of the sarcolemma that enable electrical impulses to spread quickly in a continuous cell-to-cell (syncytial) fashion. The intercalated disks contain three junctions: desmosomes, or macula adherens; the fascia adherens, which mechanically attach one cell to another; and gap junctions, also known as tight junctions or the nexus, which allow the electrical impulse to spread from cell to cell (see Chapter 1). Changes in the function of these junctional elements have been associated with an increased risk of dysrhythmias.9
The third major difference between cardiac and skeletal muscle cells involves the T tubule system. Cardiac fibers contain more T tubules than skeletal muscle fibers. This increased proximity to the T tubules gives each myofibril in the myocardium faster access to molecules it needs for the continuous transmission of action potentials, a process that involves transport of sodium and potassium through the walls of the T tubules. (The mechanisms by which sodium and potassium transport causes transmission of cardiac action potentials are described in Chapters 1 and 43.) Because the T tubule system is continuous with the extracellular space and the interstitial fluid, it facilitates the rapid transmission of electrical impulses from the surface of the sarcolemma to the myofibrils inside the fiber. This activates all the myofibrils of one fiber simultaneously. The sarcoplasmic reticulum is located around the myofibrils. When an action potential is transmitted through the T tubules, it induces the sarcoplasmic reticulum to release its stored calcium, which activates the contractile proteins, actin and myosin, within the sarcomere at the same time. T tubule loss, disruption, and dysregulation are associated with heart failure and atrial fibrillation.14
Actin, Myosin, Troponin-Tropomyosin Complex, and Titin
Within each myocardial sarcomere the thick filaments of myosin constitute the central dark band called the anisotropic, or A, bands (Figure 31-13). The myosin molecule resembles a golf club with two large bulbous heads protruding from one end of a straight shaft (Figure 31-14). The bilobed heads contain an actin-binding site and a site of ATPase activity. A thick filament called myosin microfilament is composed of about 200 myosin molecules bundled together with outward-facing molecule heads called cross-bridges because with activation of contraction they will form force-generating bridges with exposed actin molecules (see Figure 31-14, B).The actin molecules are part of the thin filaments (Figure 31-15). The light bands are called isotropic, or I, bands (see Figure 31-13). The thin filaments of actin appear light and extend from the Z line, a dense fibrous line that crosses the center of each I band. A sarcomere is the area from one dark Z line to an adjacent Z line with a length that varies from 1.6 to 2.2 mm. In the center of a sarcomere is the H zone, a somewhat less dense region. A thin, dark M line travels the center of the H zone. A single tropomyosin molecule (a relaxing protein) lies alongside seven actin molecules. Troponin, another relaxing protein, associates with the tropomyosin molecule, forming the troponin-tropomyosin complex (see Figure 31-15). The troponin complex itself has three components. Troponin T aids in binding of the troponin complex to actin and tropomyosin; troponin I inhibits the ATPase of actomyosin; and troponin C contains binding sites for the calcium ions involved in contraction. Troponin and tropomyosin are referred to as relaxing proteins because when they cover the myosin-binding sites on the actin, the cross-bridges release and the myocardium relaxes. Also within the sarcomere is a giant elastic protein called titin (or connectin), which acts as a spring and is one of the factors responsible for myocardial stiffness and thus impacts diastolic filling of the myocardium.15
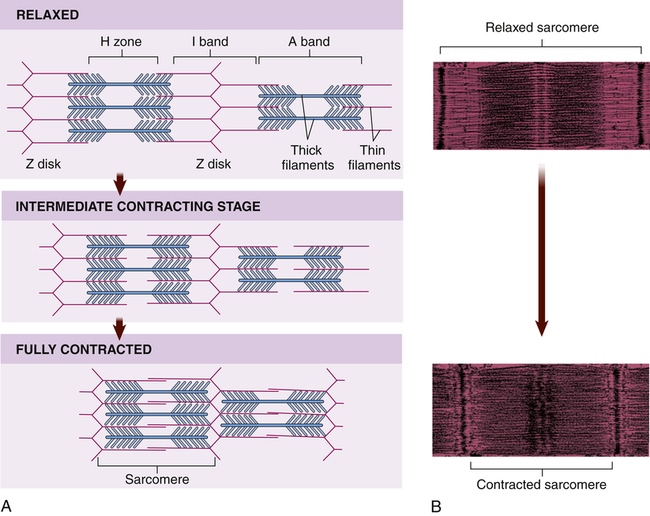
A, During contraction, myosin cross-bridges pull the thin filaments toward the center of each sarcomere, thus shortening the myofibril and the entire muscle fiber. B, Color-enhanced transmission electron micrographs (TEMs) showing the shortening of a sarcomere caused by the sliding of filaments during muscle contraction. (A from Patton KT, Thibodeau GA: Anatomy & physiology, ed 8, St Louis, 2013, Mosby. B courtesy H.E. Huxley, Brandeis University, Waltham, MA.)
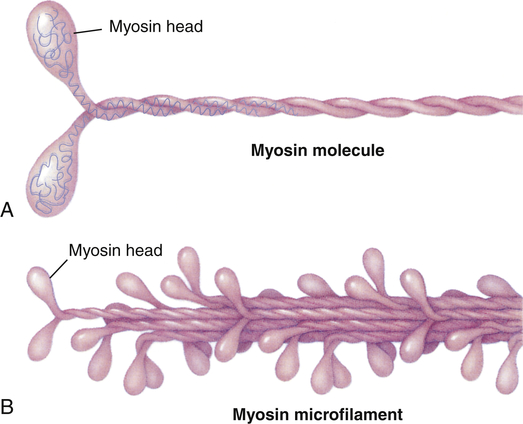
A, Each myosin molecule is a coil of two chains wrapped around each other. At the end of each chain is a globular region, much like a golf club, called the head. B, Myosin molecules usually are combined into filaments, which are stalks of myosin from which the heads protrude at regular intervals.
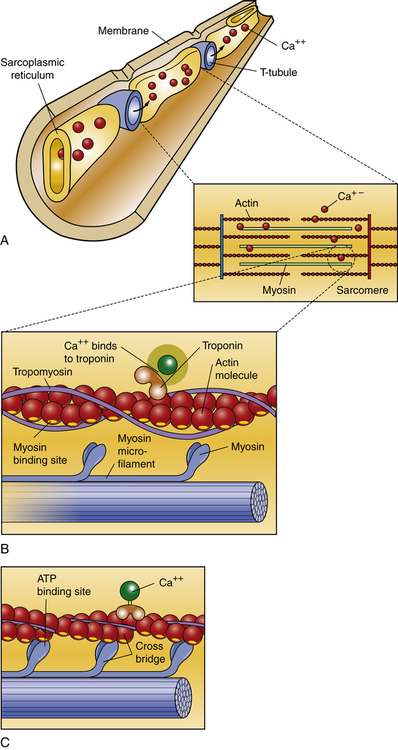
A, Thin and thick myofilaments. In resting muscle, calcium ions are stored in the sarcoplasmic reticulum. When an action potential reaches the muscle cell, the T tubules carry the action potential deep into the sarcoplasm. The action potential causes the sarcoplasmic reticulum to release the store of calcium ions. B, In resting muscle the myosin-binding sites are covered by troponin and tropomyosin. The calcium ions released into the sarcoplasm as a result of the action potential bind to the troponin. This binding causes the tropomyosin and troponin to move out of the way of the myosin-binding sites, leaving the myosin heads free to bind to the actin microfilament. C, ATP is used as an energy molecule to the myosin cross-bridge. (Adapted from Raven PH, Johnson GB: Understanding biology, ed 3, Dubuque, IA, 1995, Brown.)
Myocardial Metabolism
Cardiac muscle, like other muscle tissue, depends on the constant production of ATP for energy. ATP is produced within the mitochondria mainly from glucose, fatty acids, and lactate. If the myocardium is inadequately perfused because of coronary artery disease, anaerobic metabolism must be used as a source of energy (see Chapter 1). The energy produced by metabolic processes is used for muscle contraction and relaxation, electrical excitation, membrane transport, and synthesis of large molecules. Normally, the amount of ATP produced supplies sufficient energy to pump blood systemically.
The oxygen supply to the myocardium is delivered exclusively by the coronary arteries. From 70% to 75% of the oxygen from the coronary arteries is used immediately by cardiac muscle, leaving little oxygen in reserve. Therefore, increased energy needs can be met only by increasing coronary blood flow. Myocardial oxygen consumption can increase several-fold with exercise and decrease moderately under conditions such as hypotension and hypothermia. As myocardial metabolism and consumption of oxygen increases, the concentration of local vasoactive metabolic factors increases. Some of these, such as adenosine, nitric oxide, and prostaglandins, dilate coronary arterioles, thus increasing coronary blood flow.16
Myocardial Contraction and Relaxation
Myocardial contractility is a change in developed tension at a given resting fiber length. In functional terms, contractility is the ability of the heart muscle to shorten. On a molecular basis, thin filaments of actin slide over thick filaments of myosin, called the cross-bridge cycle of muscle contraction.17 It is the cycling of cross-bridges that is the molecular basis for myocardial force generation and thus ultimately the heart’s ability to pump blood through the circulation. Anatomically, contraction occurs when the sarcomere shortens, causing adjacent Z lines to move closer together (Figure 31-16). The width of the A band, which contains the thick myosin filaments, is unchanged while the I band becomes narrower as the overlap between the thick and thin filaments increases. The degree of shortening of the muscle fibers depends on how much the thin filaments overlap the thick filaments. Maximal contraction occurs when the sarcomere length is 2.2 mm. At 2.2 mm the number of cross-bridge attachments between actin and myosin is maximal.
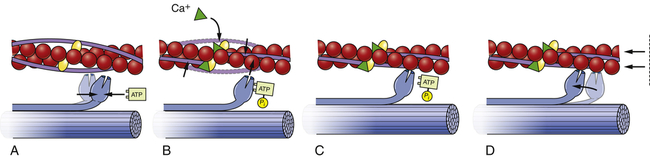
A, Each myosin cross-bridge in the thick filament moves into a resting position after an adenosine triphosphate (ATP) molecule binds and transfers its energy. B, Calcium ions released from the sarcoplasmic reticulum bind to troponin in the thin filament, allowing tropomyosin to shift from its position blocking the active sites of actin molecules. C, Each myosin cross-bridge then binds to an active site on a thin filament, displacing the remnants of ATP hydrolysis—adenosine diphosphate (ADP) and inorganic phosphate (Pi). D, The release of stored energy from step A provides the force needed for each cross-bridge to move back to its original position, pulling actin along with it. Each cross-bridge will remain bound to actin until another ATP molecule binds to it and pulls it back into its resting position (A). (Adapted from Thibodeau GA, Patton KT: Anatomy & physiology, ed 4, St Louis, 1999, Mosby.)
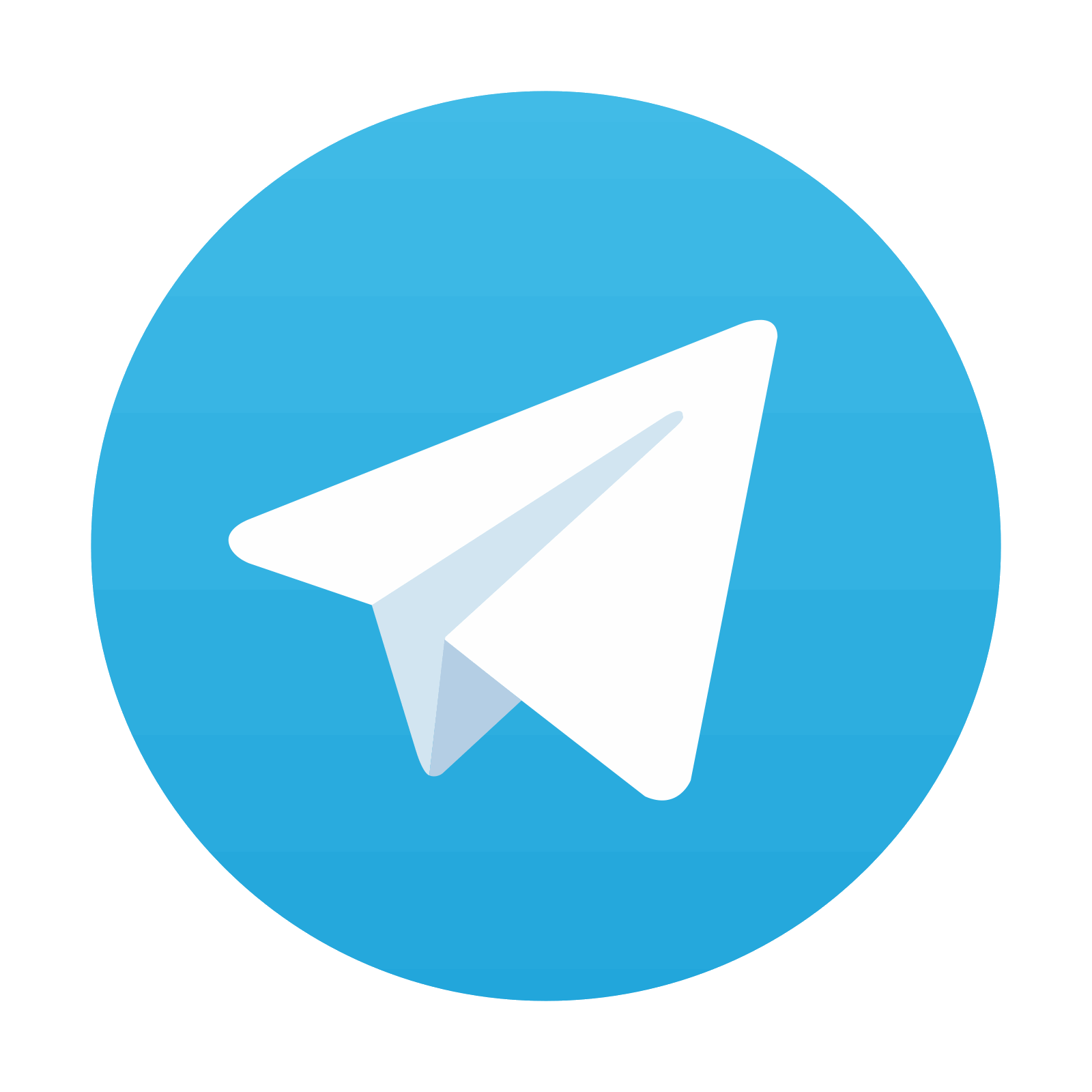
Stay updated, free articles. Join our Telegram channel
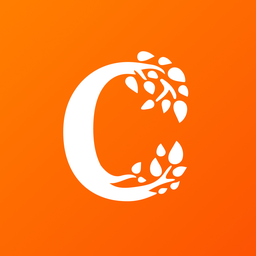
Full access? Get Clinical Tree
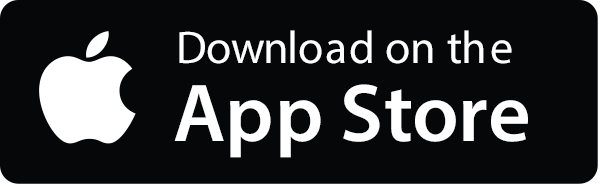
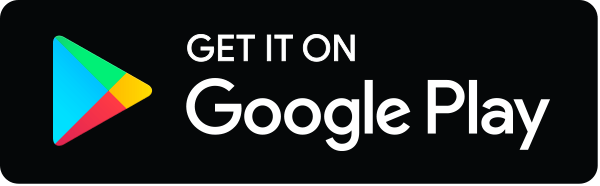