A. GENERAL OBSERVATIONS
The generation and storage of energy is one of the most important challenges of our time. The reasons include the looming crisis in the supply of oil and natural gas and the effects of burning hydrocarbon fuels on the climate of the whole planet. Solutions to these problems include electricity generated from solar cells, windmills, tides, or nuclear fission, together with the replacement of hydrocarbon fuels by hydrogen, methanol, or ethanol.
One of the most appealing solutions for both stationary and mobile power generation is the fuel cell (Figure 12.1)—a device that converts fuel directly into electricity with a higher efficiency than do methods that depend on the combustion of coal or hydrocarbons. However, fuel cells are still in a developmental stage, with hurdles to be overcome that involve mainly the limitations of existing materials. Thus, the search for new materials for fuel cells provides an excellent example of the way in which materials science holds the key to solving a crucially important problem. A critical component of a fuel cell is the material that conducts ions from one electrode to the other. The performance of most current fuel cells is severely restricted by the limitations of the available ion-conducting materials.
Figure 12.1. Essential differences between a fuel cell and a battery. A fuel cell provides electric current as long as the fuel is supplied. A battery can yield current only until its chemical fuel is exhausted. After that it must be recharged or discarded.

Power generation is not the only challenge facing the modern world. A related problem is the storage of power, particularly for use in mobile applications such as electric automobiles, laptop computers, cellular phones, and medical devices. The main method for the storage of electric power is the use of primary and secondary batteries. A primary battery yields its energy by consuming a reactive metal, while a secondary battery uses a reversible electrochemical reaction that utilizes electricity for the recharge cycle. For practical reasons, the storage of significant amounts of power requires the use of rechargeable batteries. Various rechargeable battery technologies are summarized in Figure 12.2. Three key requirements are to reduce the weight of batteries, increase the amount of energy stored per unit weight (energy density), and increase the amount of power that can be released in a given time. For some applications, resistance to failure under harsh conditions is also an important requirement. Conventional batteries use liquid electrolytes such as sulfuric acid or flammable organic solvents. This adds weight, makes them unnecessarily fragile, and in some cases creates a fire hazard. Batteries that use solid membrane electrolytes offer many advantages for the future, but here again the materials properties of the available solid electrolytes are inadequate for widespread use. In this chapter we examine some of the challenges and possible solutions to battery materials and to fuel cell membranes.
Finally, electrical energy can also be stored in capacitors. Traditionally, small capacitors are key components of electric circuits in radios, television sets, and microcomputers. However, very large capacitors could, in principle, store significant amounts of energy. The development of new materials for this purpose is another subject of growing importance.
1. Background
Fuel cells are an alternative to both heat engines and batteries for numerous applications. A comparison of the principles of batteries and fuel cells is shown in Figure 12.1. The key characteristic of a fuel cell is that it generates electrical power as long as a chemical fuel is pumped into the device. Moreover, compared to an internal-combustion engine or a steam-driven power station, a fuel cell has fewer moving parts, generates less noise and far less pollution, and can utilize a variety of fuels. The fuels may be hydrogen, methanol, or hydrocarbons that are converted by catalytic reactors to water and/or carbon dioxide, depending on the type of cell.
2. General Principles
A fuel cell is an electrochemical device that takes a reaction that would normally be highly exothermic, and moderates that process to generate electricity as well as heat. Example processes are the oxidation of hydrogen, methane, gasoline, or methanol, all of which, under normal oxidative chemical reaction conditions, would generate a fire or explosion. Note that a single fuel cell is only rarely sufficient to generate the required electrical power, and stacks of multiple cells are normally needed for practical applications.
There are many different designs for fuel cells. However, five main types of fuel cell are already in use or are under development: (1) the polymer electrolyte membrane (PEM) fuel cell, (2) the phosphoric acid electrolyte cell, (3) the alkaline fuel cell, (4) the molten carbonate fuel cell, and (5) the solid oxide system. The first two function through the presence of a proton-conducting material that separates the anode from the cathode. The remaining three use chemistry that involves the transport of anions from the cathode to the anode. The main differences between these units are (1) the type of electrolyte, (2) the temperature of operation, (3) the type of metal catalysts that are needed to facilitate the reactions that occur at the electrodes, and (4) the degree to which the different designs can tolerate impurities in the fuel supply. These five systems are summarized in Table 12.1, and are discussed in turn.
3. Polymer Electrolyte Membrane (PEM) Fuel Cells
The main driving force for the development of polymer electrolyte fuel cells is their suitability for operation at moderately low temperatures and for mobile applications such as in automobiles and portable devices such as cellular phones or laptop computers. Unlike internal combustion engines, they produce power without the formation of nitrogen oxide pollutants. A cross section of a polymer electrolyte membrane fuel cell, together with the reactions involved, is shown in Figure 12.3.
A PEM fuel cell consists of two electrodes that contain finely divided precious-metal catalyst particles separated by a proton conducting polymer membrane. The anode typically is constructed from carbon fiber cloth and/or powdered graphite, with platinum or platinum–ruthenium alloy particles covering the surface of the carbon. The purpose of the anode is to convert the fuel to protons and electrons at a temperature of 80°C or higher. When the fuel is a hydrocarbon such as methane, a catalytic reactor is positioned ahead of the fuel cell to convert the hydrocarbon to hydrogen, carbon dioxide, and perhaps carbon monoxide. If hydrogen is the fuel entering the fuel cell anode, the only products are protons and electrons. When methanol is the fuel, a catalyst at the anode converts it to carbon dioxide, protons, and electrons. In both cases the electrons pass through the external circuit and eventually reach the cathode.
Figure 12.3. Schematic cross section of a direct hydrogen or direct methanol polymer electrolyte membrane (PEM) fuel cell.

TABLE 12.1. Characteristics of Different Types of Fuel Cells

aFuel actually entering the device. Many fuel cells require a “preconverter” to change methane or gasoline to hydrogen and carbon dioxide. Fuel cells that use line hydrogen are dependent on off-site conversion of methane or higher hydrocarbons to hydrogen and carbon dioxide, or the reaction of carbon (coke) with water (the water–gas shift reaction) to a mixture of hydrogen, carbon monoxide, and carbon dioxide.
The cathode is also typically constructed from carbon fiber cloth or particles, with both infused with platinum particles. At the cathode, the protons that have traversed the membrane react with both oxygen from air and the incoming electrons to form water, again at 80°C or higher temperatures.
Not shown in Figure 12.3 are the metal or graphite gas distribution channels that funnel the fuel and air evenly into the porous anode and cathode.
The most critical part of a polymer electrolyte fuel cell is the proton-conducting membrane, which serves two purposes; it must (1) conduct protons from the anode to the cathode and (2) not allow the fuel (hydrogen or methanol) to pass through to the cathode. If the fuel does reach the cathode, it will be oxidized without generating an electric current. Proton conduction in a polymer electrolyte membrane is achieved by the linkage of acidic functional groups, such as sulfonic acid (SO3H) groups, to a polymer. The acidic groups provide “way stations” for protons to be transferred from one acidic site to another. A cartoon showing how this may occur is shown in Figure 12.4.
Sulfonic acid groups are the most commonly used acidic units, although experimental membranes with phosphonic acid or sulfonimide units are also being studied. These membranes are called “single-ion conductors” because only the protons are able to move. The anions, such as the sulfonate, or phosphonate ions are covalently linked to the polymer chains of the membrane, and are thus immobilized.
The design, synthesis, and fabrication of a suitable proton-conducting membrane is a major challenge in this technology. Part of the problem is the need for membranes that function effectively at temperatures above 80°C, because higher temperatures allow the use of less expensive electrode catalysts that are less sensitive to catalyst poisoning. High temperatures also accelerate the movement of protons from anode to cathode. Unfortunately, most of the existing polymer electrolyte materials make use of water trapped in the membrane to facilitate the transport of protons, and this water is lost at temperatures above 80–90°C. Example polymers that have been or are being investigated for fuel cell membranes are shown as species 12.1–12.8

Figure 12.4. Proton migration through an amphiphilic membrane is believed to occur through water-rich channels by transfer from one acidic site to another. Each proton moves accompanied by a solvent sheath of water molecules. The higher the loading of SO3H groups, the higher will be the proton conductivity, but the more the membrane will swell by absorbing water. This hydrophilicity must be balanced by hydrophobic regions and/or by crosslinking the chains to give mechanical strength to the membrane.



a. Nafion.
Nafion 117® is the classical proton-conductive fuel cell membrane. It is a fluorocarbon polymer with sulfonic acid groups connected to the structure. A representation of the structure of Nafion is shown as structure 12.1. This polymer consists of a hydrophobic part (the fluorocarbon) and a water-absorbing hydrophilic part (the sulfonic acid groups), which phase-separate to generate water-containing hydrophilic channels through which the protons diffuse. The exact mechanism of proton conduction is still a subject for debate. However, it seems clear that the protons generated at the anode move through the membrane from one acidic site to another, assisted by the shell of water molecules that accompanies each proton. Thus, when the water is lost by evaporation at temperatures above ~80°C, the proton conduction ceases. The hydrophobic fluorocarbon domains are probably needed to provide strength to the membrane since they cannot absorb water. It is possible to operate Nafion-based fuel cells using elevated pressures to retard water loss, but this increases the engineering complexity of the system.
Although Nafion prevents transport of molecular hydrogen across the membrane, it has a high affinity for and is highly permeable to methanol. Thus, methanol crosses the membrane and is oxidized at the cathode without the production of electric power. For this rerason, Nafion is unsuitable for the small portable direct methanol fuel cells that are needed to power laptop conputers, cellular phones, or even automobiles that use methanol as a fuel. Use of dilute solutions of methanol in water reduces methanol permeation but seriously decreases the efficiency of the process.
Nafion is an example of a single-ion conductor, in which protons are free to move but the anionic sulfonate groups are immobilized on the polymer.
b. Sulfonated Polystyrene (Structure 12.2).
Sulfonated polystyrene was one of the earliest and simplest fuel cell membranes. It was used in the Gemini space mission. This material was employed in a pure hydrogen/oxygen fuel cell. It had a satisfactory performance over the short time-period of the mission, but suffered from oxidative degradation of the polymer during extended operation.
c. Sulfonated Polyimides.
These are complex condensation polymers with some combination of the repeating units shown in structure 12.3. They are thermally stable, their mechanical properties can be varied by changes to the ratio of different repeating units and sulfonic acid groups, they can be used for both hydrogen and direct methanol fuel cells, and they can be crosslinked to provide membrane strength. The disadvantage is the complexity of synthesis.
d. Sulfonated Poly(phenylene oxide) (Structure 12.4).
Poly(phenylene oxide) is an inexpensive, highly stable condensation polymer that can be sulfonated readily. The ratio of hydrophilic sulfonate groups to hydrophobic units is controlled by the degree of sulfonation. The methanol permeability is low.
d. Polyphosphazenes with Acidic Functional Groups (Structures 12.5–12.7).
The electrochemical stability of the polyphosphazene backbone offers some advantages for fuel cell applications. Most research with these polymers has utilized macromolecules that bear aryloxy side groups to which are linked acidic units such as sulfonic acid, phosphonic acid, or sulfonimide functional groups. Typical examples are shown in structures 12.5–12.7. Because membranes derived from these polymers show resistance to methanol crossover, the promise for them lies mainly for uses in direct methanol fuel cells.
F. Poly(benzimidazoles) (Structure 12.8).
Attempts are being made to combine the attributes of proton donors such as phosphoric acid (see the following section) with the solid properties of polymer membranes. For example, poly(benzimidazole) copolymers such as structure 12.8 can be “doped” with phosphoric acid or other acids to allow proton conduction at elevated temperatures in the absence of water. The mechanism of proton transfer is believed to be via a hopping of protons from the lone-pair electrons of one skeletal nitrogen atom to another, a process that eliminates the need for water. However, the oxidation reaction at the cathode generates water, and some concern exists that this water may extract the phosphoric acid over long periods of fuel cell operation.
Numerous other polymers have been synthesized and evaluated for fuel cell applications. Some of these, such as structures 12.3–12.8, are better than Nafion at resisting methanol crossover. However, few of the water-containing fuel cell membranes function well above 80–100°C except when the device is operated under sufficient pressure to prevent the volatilization of water. This is clearly an area of keen interest for future energy generation devices.
4. Phosphoric Acid Fuel Cells
One of the most highly developed fuel cells for stationary applications, such as in office buildings, hospitals, and small power stations, is the phosphoric acid fuel cell. Its design and mode of operation are shown in Figure 12.5.
The fuels are hydrogen and oxygen. The electrodes are typically carbon in the form of carbon fiber “paper” or some other porous modification of this material. Like the PEM fuel cell described above, molecular hydrogen enters the device at the anode, where it is converted to protons and electrons by a catalyst such as platinum. However, the transport of protons to the cathode takes place through a liquid electrolyte of molten phosphoric acid. At the cathode, protons and the electrons coming in through the external circuit react with oxygen from the air to produce water.
The overall efficiency of a phosphoric acid fuel cell is high (>40%). Moreover, the system is relatively insensitive to impurities such as CO2 in the fuel. Molten phosphoric acid is a preferred electrolyte because of its low volatility and stability. However, the proton mobility in phosphoric acid at room temperature is low, and the electrolyte must be heated to temperatures near 200°C before satisfactory conductivities can be achieved. A deterrent for the use of these devices in mobile applications used by the general public is the corrosive characteristic of the molten acid, which would constitute a serious hazard. For stationary applications, a significant advantage is the production of high-temperature steam as a byproduct. This can be used for heating buildings or driving a turbine for supplementary electricity generation. This supplementary energy production raises the overall energy efficiency into the range of 80%.
5. Alkaline Fuel Cells
The principle of operation for alkaline fuel cells is different from that for the two examples described above in the sense that they depend on the transport of anions from the cathode to the anode. The electrolyte is an aqueous solution of potassium hydroxide in an inert porous matrix instead of an ion conductive polymer or molten acid. The electrodes are constructed from a chemically inert material such as carbon fiber (Chapter 7) or porous carbon. The fuels are hydrogen and oxygen, and the products are water and electrical power. A schematic of an alkaline fuel cell is shown in Figure 12.6.
Figure 12.6. An alkaline fuel cell, which has many advantages for spaceflight, uses a liquid electrolyte of potassium hydroxide in water, but requires the use of highly purified hydrogen and oxygen as fuels.

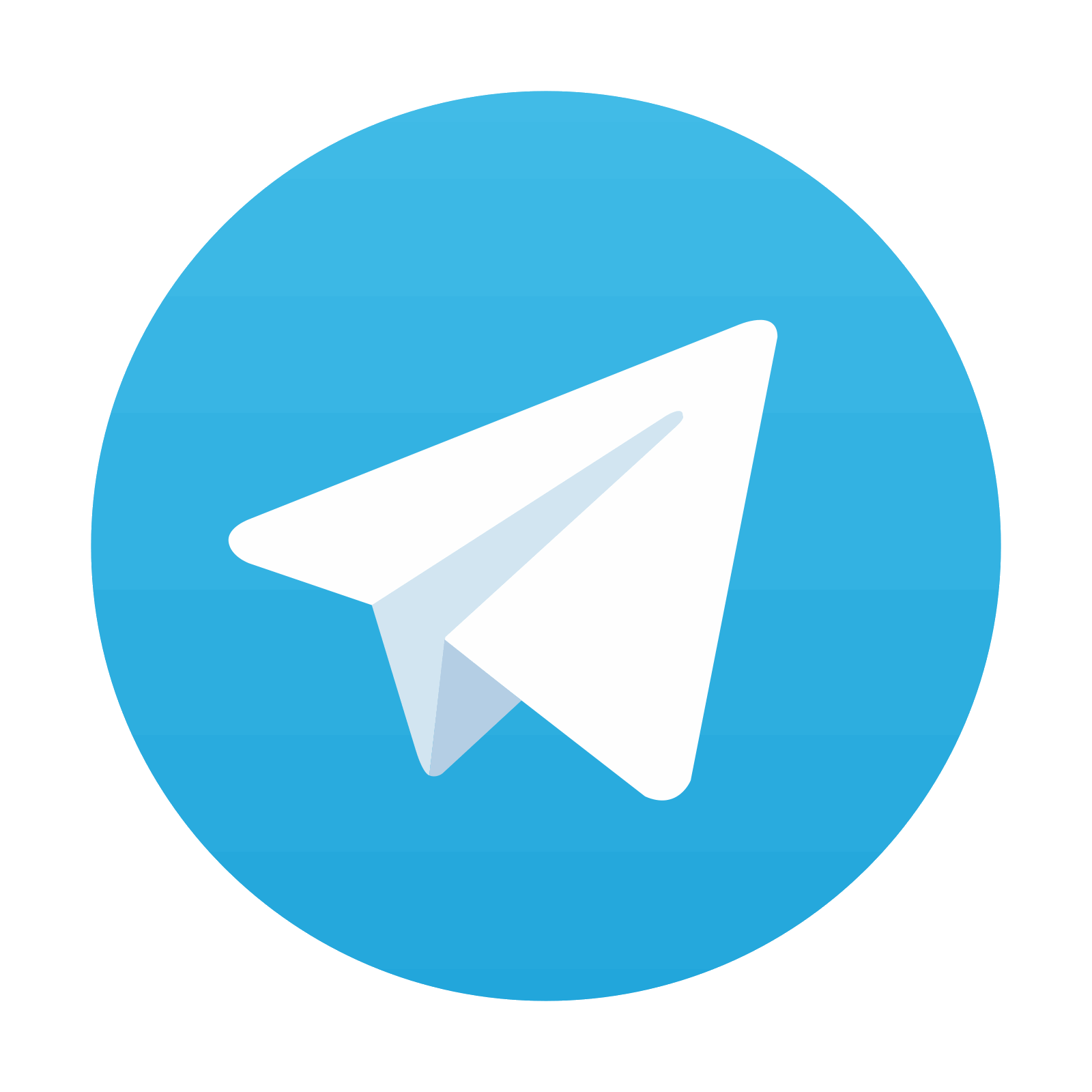
Stay updated, free articles. Join our Telegram channel
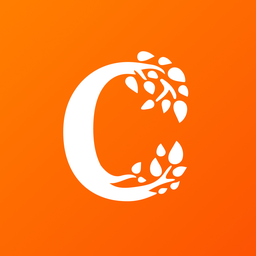
Full access? Get Clinical Tree
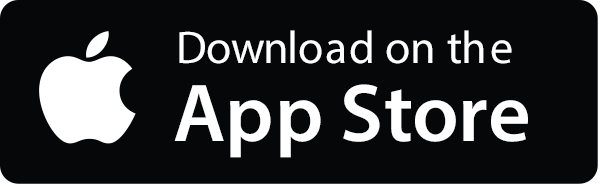
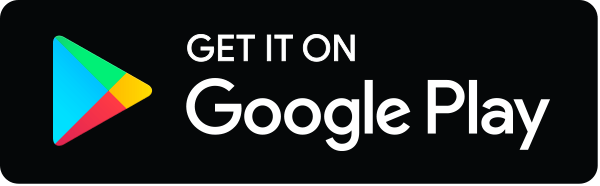