A. IMPORTANCE OF SMALL-MOLECULE MATERIALS
Small molecules in solids play an important role in electronics, photonics, and molecular separations. Small molecules can become assembled in the solid state in several different ways, all of which provide a useful introduction to the behavior of the more complex materials discussed in later chapters.
First, small molecules often become packed into a highly ordered crystalline three-dimensional lattice. Such crystalline solids are among the simplest and easiest materials to understand, and they can be studied with great accuracy by single-crystal X-ray diffraction techniques (see Chapter 4). This self-assembly of small molecules into an ordered three-dimensional array may occur not only when the molecules crystallize from solution or from the melt but also when they are condensed directly into the solid state from the vapor.
Second, the formation of crystals from solution or from molten mixtures may yield crystalline solids that contain more than one small-molecule species. These can involve anion/cation or charge transfer associations, or they may be solids in which neutral guest molecules occupy space between the host molecules. These last systems are formed from molecules that, because of their shape, crystallize with significant empty space (free volume) in the lattice, into which small “guest molecules” can fit. They are called clathrates or “molecular inclusion compounds,” although they are not “compounds” in the usual sense of the word.
Third, small molecules in solids may exist in the form of an amorphous glass, in which the molecules are oriented randomly. This situation is less common for small molecules than it is for polymers or ceramics (see Chapters 6 and 7), but it can occur with small molecules if a molten liquid is cooled rapidly, and if the molecules are highly flexible or have irregular shapes.
Finally, within a discrete temperature range, small molecules sometimes occupy a phase that is intermediate between a crystalline or amorphous solid and a liquid—the so-called liquid crystalline state.
B. PACKING OF SMALL MOLECULES IN THE SOLID STATE
The packing arrangements of small molecules in a crystal are determined mainly by three factors—shape-fitting, molecular dipoles or charges, and hydrogen bonding. These are considered in turn.
1. Shape-Fitting
Figure 5.1 illustrates the principles involved in shape-fitting. Neutral molecules attempt to assume a minimum energy orientation in terms of the attractions and repulsions between them and their near neighbors. Thus, van der Waals–type forces (see Chapter 2) are the main influence on the shape-fitting process, and the separation between adjacent molecules and their orientation relative to each other are a result of this energy minimization. Occasionally more than one packing arrangement will be acceptable, and this is responsible for crystal structure changes as the solid is heated, cooled, or subjected to pressure.
It is possible to calculate the minimum–energy packing arrangement using known or predicted interaction parameters such as a 6:12 Lennard-Jones function (again see Chapter 2), but in practice it is usually quicker to carry out a single-crystal X-ray diffraction study and examine the experimentally determined packing arrangement.
Small molecules come in so many different shapes that it is not possible to generalize about shape-fitting. Nevertheless, certain well-known examples exist that provide a starting point for understanding the relationship between molecular shape and solid-state structure. As shown in Chapter 2 (Figure 2.12), disk-shaped molecules frequently stack in the solid state like plates or saucers. Fused aromatic ring systems in the solid state often assume a tilted stack arrangement. The same is true of phthalocyanine molecules.
Figure 5.1. A simple classical example of shape-fitting, showing how a motif in the form of hexamethylbenzene can be assembled on a lattice to generate a two-dimensional array that minimizes the free volume in the crystal. (From Bunn, C. W., Chemical Crystallography , Oxford University Press, 1963.)

Short-chain linear aliphatic organic molecules assume a zigzag conformation and pack closely as shown in Figure 2.12. Some paddle-wheel-shaped molecules may pack in a way that interleafs their “paddles” or in a more open structure that generates cavities or tunnels. Examples of these possibilities are described later. Spherical molecules such as fullerenes assemble in the solid state much like metal atoms since there are relatively few alternative packing arrangements for spheres.
2. Dipolar or Charged Molecules
Small molecules with dipoles or separated charges attempt to achieve minimum-energy packing arrangements by bringing the positively charged regions close to the negatively charged parts of neighboring molecules (Figure 5.2). In practice, the crystal structure is the result of some compromise between charge neutralization and shape fitting, and is difficult to predict by modeling techniques. Here again, an X-ray structure is usually the fastest way to understand how these factors achieve a balance.
Note that there is an important difference between the packing of uniatomic ions in solids and the packing of charged molecules. The ions are spherical, and the variations in packing opportunities are far fewer than in nonspherical molecules.
3. Hydrogen Bonding
The crystal structures of molecules such as amino acids, hydroxycarboxylic acids, dicarboxylic acids, phenols, amines, or water may be strongly influenced by the opportunities for hydrogen bonding. Hydrogen bonds, although weak by comparison with covalent or coordinate bonds, nevertheless exert a strong influence on packing because of the proximity of functional groups in the solid state and the limited molecular motions that are possible in a solid. Water ice is a well-known example of a hydrogen bonded solid, and this system is described later.
Figure 5.2. Molecules with dipoles or charged ends may become organized in the solid state in a manner that brings opposing charges into close proximity.

C. SELF-ASSEMBLY BY CRYSTALLIZATION
One of the extraordinary aspects of solid-state chemistry and physics is the way in which small molecules in solution or in the molten state assemble at the surface of a growing crystal and select a minimum-energy orientation. Considerable sampling of different orientations must take place at the interface, augmented by the need to conform to the crystal structure on the existing surface. This is why seed crystals are sometimes needed to initiate crystal growth, and why crystallization of a new compound may be difficult until nano- or microsized crystals are present in the laboratory to initiate the crystallization process. It is also interesting that impurity molecules are usually excluded from the crystal because of the forces that favor the deposition of molecules in an orientation that matches those that already exist at the surface. Shape-fitting or polar forces undoubtedy explain this behavior. The exclusion of impurity molecules underlies the common laboratory practice of purification of reaction products by recrystallization. The purification of silicon for semiconductor applications is critically dependent on this phenomenon (see Chapter 10).
Another aspect of template-induced crystallization is the deposition of molecules from the vapor state on to a crystalline surface. The crystal structure of the deposited material may be controlled by the structure of the substrate.
D. SPHERICAL MOLECULES SUCH AS FULLERENES IN THE SOLID STATE
Perhaps the simplest mode of molecular packing in the solid state is provided by C60 fullerene (“buckyball”) molecules. These spherical molecules pack into a crystal lattice in the manner described elsewhere for ions or metal atoms. In other words, because of the spherical shape, the assembly pattern is determined by the most efficient way to assemble spheres in three dimensions, like a collection of ping-pong balls.
Figure 5.3a shows the crystal packing arrangement of C60 at room temperature. It is a face-centered cubic arrangement, which means that it can be visualized by assembling a first layer of spheres, with each one in contact with six others. The second layer is constructed from spheres placed in the depressions in the first layer, and the third layer is formed from spheres placed in the depressions in the second layer. Successive layers then repeat this sequence. At ambient temperature the spheres undergo rotation or tumbling in place within the lattice. However, the presence of metal atoms such as potassium trapped in the remaining space in the lattice, as in M3C60, yields a solid that is a room-temperature electronic con ductor, but becomes a superconductor when cooled below 18 K (see Chapter 11). The electrical conductivity is a consequence of electrons being transferred from the metal atoms to the π orbitals of fullerene molecules, and, because the spheres are in close contact, the electrons are free to move from molecule to molecule. The face-centered cubic (FCC) structure can accommodate no more than three metal atoms per C60 molecule (Figure 5.3b), and the presence of six metal atoms, as in M6C60, results in a reorganization of the lattice and a loss of electrical conduction.
Figure 5.3. (a) Packing of C60 molecules in a face-centered cubic (FCC) lattice. (b) Incorporation of alkali metal cations (small closed circles) into the octahedral spaces in the C60 lattice. A ratio of three cations to each C60 allows the original FCC lattice to be retained and gives electrical conductivity. A higher ratio of cations causes the additional ions to occupy tetrahedral holes in the lattice, and that structure is nonconductive.

E. DISK-SHAPED MOLECULES AND OTHER FLAT STRUCTURES
Many disk-, ribbon- or wafer-shaped organic molecules exist. Examples include benzene and symmetric trisubstituted benzenes, triphenylene, coronene, and phtha-locyanines (see Figure 5.4). Ribbon- or wafer-shaped structures such as tetracene and pentacene are related species.
A characteristic of many disk- or ribbon-shaped molecules is that they tend to self-assemble in a way that generates columns of stacked disks or short ribbons. The molecules in each stack may be oriented at right angles to the axis of the stack like a pile of plates or saucers, or they may be tilted (see Figure 2.12). A stacked packing arrangement provides opportunities for two phenomena to appear—discoid liquid crystalline behavior, or the possibility of electron movement down a stack. These are considered in turn.
1. Liquid Crystallinity from Disk- or Wafer-Shaped Molecules
Liquid crystallinity is a phenomenon that occurs when a solid is heated but does not undergo a clean transition from solid to liquid at the melting point. Instead, an intermediate phase (a mesophase) is formed that retains some of the order associated with the solid state but has fluidity more like that in the molten state. Similarly, if the molten material is cooled, it may not solidify at the melting point but will form a liquid-like phase with some crystalline-type association between individual molecules. The liquid crystalline phase is important because it allows molecules to be switched from one orientation to another by means of an electric field (Chapter 14). The true crystalline solid state does not allow this to happen, and the isotropic molten state permits too much random reorientation to be useful. Several quasi-disk-shaped molecules, such as cholesteryl benzoate (Figure 5.5), form liquid crystalline phases. However, the most heavily studied liquid crystalline systems are based on rod-shaped molecules (see later).
Figure 5.4. Examples of disk- or ribbon-shaped molecules that self-assemble in the solid state to form stacked structures: (a) phthalocyanine; (b) metallophthalocyanines; (c) substituted benzene molecules; (d) triphenylene; (e) coronene; (f) tetracene; (g) pentacene.

The stacking of disk- or wafer-like molecules encourages liquid crystalline behavior because the van der Waals cohesive forces that bind molecules in a stack may be stronger than those that bind adjacent columns. Thus, columns may separate from each other at a certain temperature, but the columns themselves or parts of columns remain intact. Even if the columns break up, the coorientation of the molecules may remain (see Figure 5.6a).
2. Electronic Phenomena from Disk-Shaped Molecules in the Solid State
These phenomena are associated with delocalized (aromatic) molecules. When such molecules are stacked in columns in the solid state, the π orbitals of one molecule overlap their counterparts in the molecules above and below in the stack. This generates an extended π system with electron delocalization extending down the whole column. Under favorable circumstances, this yields a semiconductor- or conductor-type band structure rather than discrete molecular orbitals (for details, see Chapter 10). However, this factor alone does not ensure electronic conductivity. Like metals, conductive columnar structures are characterized by the presence of unfilled highest-energy states in the valence band, a feature that allows holes or electrons to move down a stack. This may be facilitated by “doping” the crystal with species that accept or donate electrons from or to each column. For example, iodine molecules accommodated between the stacks can accept electrons to form, for example, . The unfilled energy levels in the host column at the top of the valence band (i.e., unpaired electrons) correspond to radical cations. This permits metal-level conductivity via fast migration of the resultant holes (positive charges) down each column. Alternatively, doping with species that readily release an electron to the stack (such as metal atoms) will yield unpaired electrons (radical anions) that may be charge carriers. Hole conduction is more common. If the dopants occupy sites between the molecules in a stack, the disks will become separated beyond the distance required for molecular orbital overlap—hence the need for dopants to be intercalated between the stacks rather than between the molecules in each stack. Doping can also be accomplished by adding or removing electrons by contact of the solid with the surface of electrodes in an electrochemical cell. With these facts in mind, we can now examine a few specific examples.
Figure 5.5. Examples of small molecules that exhibit liquid crystalline behavior in the molten or solution state.

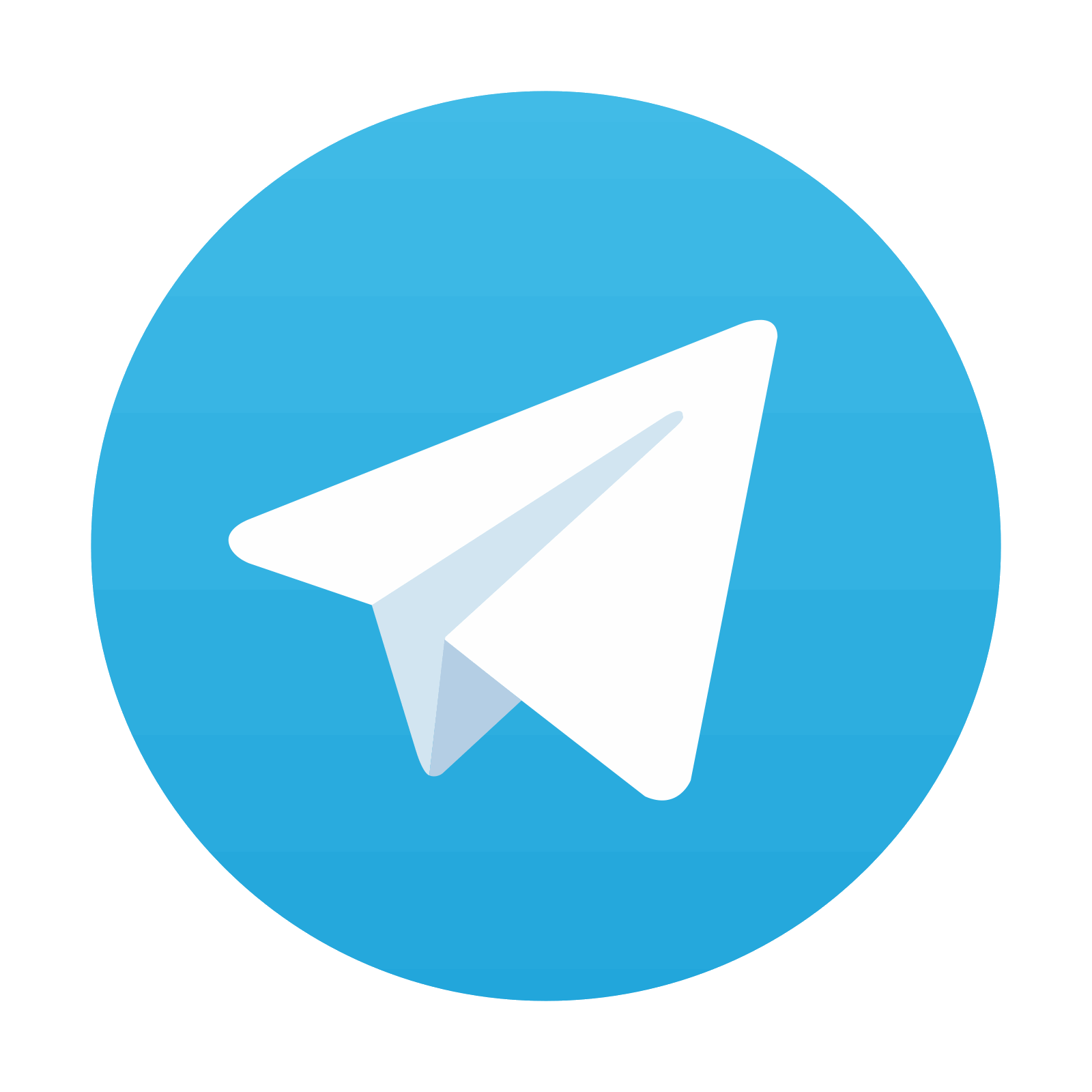
Stay updated, free articles. Join our Telegram channel
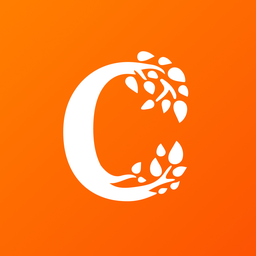
Full access? Get Clinical Tree
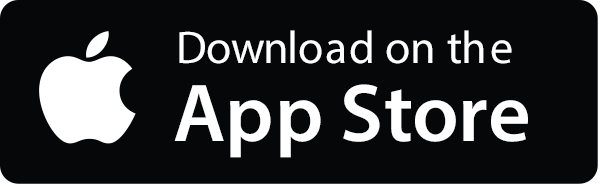
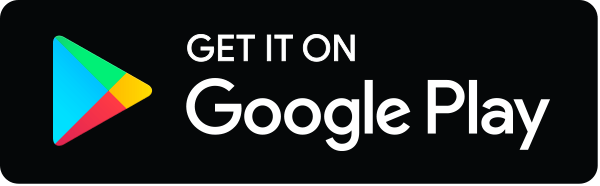