A. OVERVIEW
Superconductors are materials that offer no resistance to the passage of an electric current. The significance of this phenomenon is profound. Without electrical resistance, a current will continue to flow indefinitely within the material, magnetic fields generated by the current will be stronger and need very little current to maintain them, and superconducting objects placed in an external magnetic field will levitate the magnet—specifically, the magnet will become suspended in the air. Applications that utilize these phenomena include strong magnets for use in NMR spectroscopy, trains that move above the track but do not touch it, high-performance electric generators and motors, and power lines that transmit much larger amounts of electric current than do conventional wires. More fanciful proposed applications include shields against strong magnetic pulses, noncontact bearings, rocket launchers, energy storage rings, and rapid-switching electronic circuits and detectors. The catch to all these applications is that no materials are known that are superconductors at room temperature. All the known examples must be cooled to temperatures that vary from near zero degrees Kelvin to 138 K, depending on the type of material.
The superconductivity of mercury at a temperature near 4 K was first reported by Onnes in 1911. Between then and 1986, roughly 30 metals, a number of simple alloys, some lead molybdenum sulfides such as PbMo6S8 known as Chevrel phases, and an unstable polymer, poly(sulfur nitride), were found to become superconductors when cooled to temperatures close to absolute zero. The maximum temperature (the transition temperature) at which superconduction could be generated during this period was 23 K. In 1986 a far-reaching discovery was made by Bednorz and Muller in Switzerland that certain ceramic derivatives produced from copper oxide plus rare-earth oxides became superconducting when cooled below 30 K. The significance of this discovery lay less in the 7 K increase in transition temperature and more in the recognition that copper oxide ceramics, with all the opportunities for synthetic variations that they provided, offered the promise of dramatic increases in the temperatures at which superconduction could be achieved. Indeed, subsequent developments in rapid succession raised the superconducting transition temperature to 138 K, a temperature that can be reached by cooling with liquid nitrogen rather than liquid helium. This “highest” temperature material contains mercury, thallium, calcium, and barium as well as copper and oxygen. Altogether more than 6000 different materials are now known to have superconducting properties, including more than 50 different copper oxide–based ceramics. Thus, these high-temperature species have been the focus of most of the recent research in this field. Some classical metallic superconductors and their superconducting transition temperatures are listed in Table 11.1.
The plot of electrical resistance versus temperature shown in Figure 11.1 illustrates the behavior of most superconductors. At room temperature they are metal-level conductors or semiconductors. The resistivity decreases only slightly as the temperature is lowered until the superconducting transition temperature Tc is reached, at which point the resistance falls sharply to zero. Provided the temperature is maintained below this point, current continues to flow through the material indefinitely, generating a strong magnetic field that can be utilized in a wide range of electrical devices.
In addition to metal alloys and cuprate ceramic superconductors, superconduction has also been detected for a polymer, poly(sulfur nitride) (polythiazyl), near absolute zero. Carbon nanotubes and fullerenes also superconduct under special conditions. Moreover, a few conventional polymers such as oxidized polypropylene show high conductuvities at room temperature—not quite superconducting but higher than metals. These are termed “ultraconductors.”
In this chapter we focus on high-temperature cuprate superconductor species in terms of (1) the nomenclature, (2) how they are synthesized, (3) solid-state structure, (4) possible mechanisms of superconduction, and (5) current and emerging applications. The other types will be mentioned briefly in the appropriate sections.
TABLE 11.1. Examples of Low-Temperature (Type 1) Metallosuperconductors
Metal | Tc (K) at Ambient Pressure |
Titanium | 0.4 |
Cadmium | 0.517 |
Osmium | 0.66 |
Zinc | 0.85 |
Molybdenum | 0.915 |
Aluminum | 1.175 |
Thallium | 2.38 |
Chromium | 3.00 |
Indium | 3.41 |
Tin | 3.72 |
Mercury | 4.15 |
Lanthanum | 4.88 |
Lead | 7.196 |
Niobium | 9.5 |
Figure 11.1. Resistance versus temperature profile for YBa2Cu3O7, showing the onset of superconductivity as the material is cooled to 90 K.

TABLE 11.2. Formulas of Several High-Temperature (Type 2) Superconductors and Their Superconducting Transition Temperatures
Formula | Tc (K) |
La1.8Sr0.2CuO4 | 35 |
TlSrLaCuO5 | 40 |
Bi2(CaSr)3Cu2O8 | 90 |
(Ba,Sr)CuO2 | 90 |
YBa2Cu3O7 | 92 |
Bi2(CaSr)4Cu3O10 | 110 |
Tl2Ca2Ba2Cu3O10 | 125 |
High-temperature superconductors have a number of different formulas, as summarized by the examples in Table 11.2.
There are two different name–abbreviation systems in use. The simplest system lists the number of elements of a certain type in the formula. For example, YBa2Cu3O7-z is a “1,2,3-type superconductor,” with the numbers simply denoting the number of metal atoms in the formula unit. The number of oxygen atoms is not specified because that number varies according to processing conditions. Using the same system, Tl2Ba2CuO6 is a 2,2,1 material, Tl2CaBa2Cu2O8 would be a 2,1,2,2 material, and Tl2Ca2Ba2Cu3O10 would be a 2,2,2,3 superconductor. This system can soon become unmanageable unless the types of metal atoms are arrayed in some previously agreed on sequence.
An alternative nomenclature is based on the crystal structure and on the number of planes within a conductive unit or block. All copper oxide superconductors contain sheets of conductive copper oxide planes that alternate with electrically insulating and spacer sheets. Thus, this naming system specifies four numbers. The first is the number of insulating layers between conducting sheets. For example, TlO layers are insulating. The second number denotes the number of spacer sheets between copper oxide sheets: BaO layers are spacers. The third number indicates the number of separating layers: CaO layers are separators. The fourth number refers to the number of CuO planes within a conductive unit or block. Thus, TlBa2Ca2Cu3O9 is a 1,2,2,3 system. Clearly, it is necessary to know the crystal structure before the material can be named. The safest system for a chemist is to simply specify the full formula.
C. SYNTHESIS OF HIGH-TEMPERATURE SUPERCONDUCTORS
The synthesis of ceramic superconductors is, in principle, a process that requires a complicated sequence of simple steps. The equipment needed is found in most chemistry, chemical engineering, or ceramics laboratories. A typical procedure for the synthesis of YBa2Cu3O7-z is as follows:
1. A finely ground mixture of yttrium oxide, barium carbonate, and copper oxide in a ratio of Y:Ba:Cu of 1:2:3 is heated in air in a furnace to ~950°C for 18–24 h in an alumina or porcelain dish. This process generates the fundamental 1,2,3 crystal structure, but with a deficiency of oxygen. The conglomerate is then cooled to room temperature and pulverized.
2. The material is heated again for several more hours with the temperature rising from 500°C to ~950°C under a flow of oxygen, and then held at ~950°C for 18 or more hours before the temperature is allowed to fall slowly (~100°C/h), again all the time under a flow of oxygen. This step increases the amount of oxygen in the solid, a process known as oxygen annealing. The material is then reground to a powder.
3. Finally, the black powder, or a pellet pressed from it, is placed in an alumina dish and is then heated to 950–1000°C for about 18 h before being allowed to cool at a rate of less than 100°C/h under oxygen. The slow rate of cooling in this step and in step 2 is critical for obtaining the best superconducting material. A crucial aspect of this procedure is providing an opportunity for oxygen to enter or leave the lattice at temperatures above 400°C. A typical heating/cooling sequence is shown in Figure 11.2.
Following the synthesis process, a good test for superconductivity is the Meissner effect. With the superconductor pellet in a container, a small rare-earth magnet is placed on top of it and liquid nitrogen as added slowly. When the temperature of the superconductor falls below its transition temperature, the magnet should rise and levitate above the superconductor. Another test is a direct measurement of conductivity. The conductivity is measured by means of a four-point probe (see Chapter 4) as the temperature is lowered by adding liquid nitrogen. As the super-conducting transition is reached, the current flow suddenly increases dramatically and the resistance falls to zero.
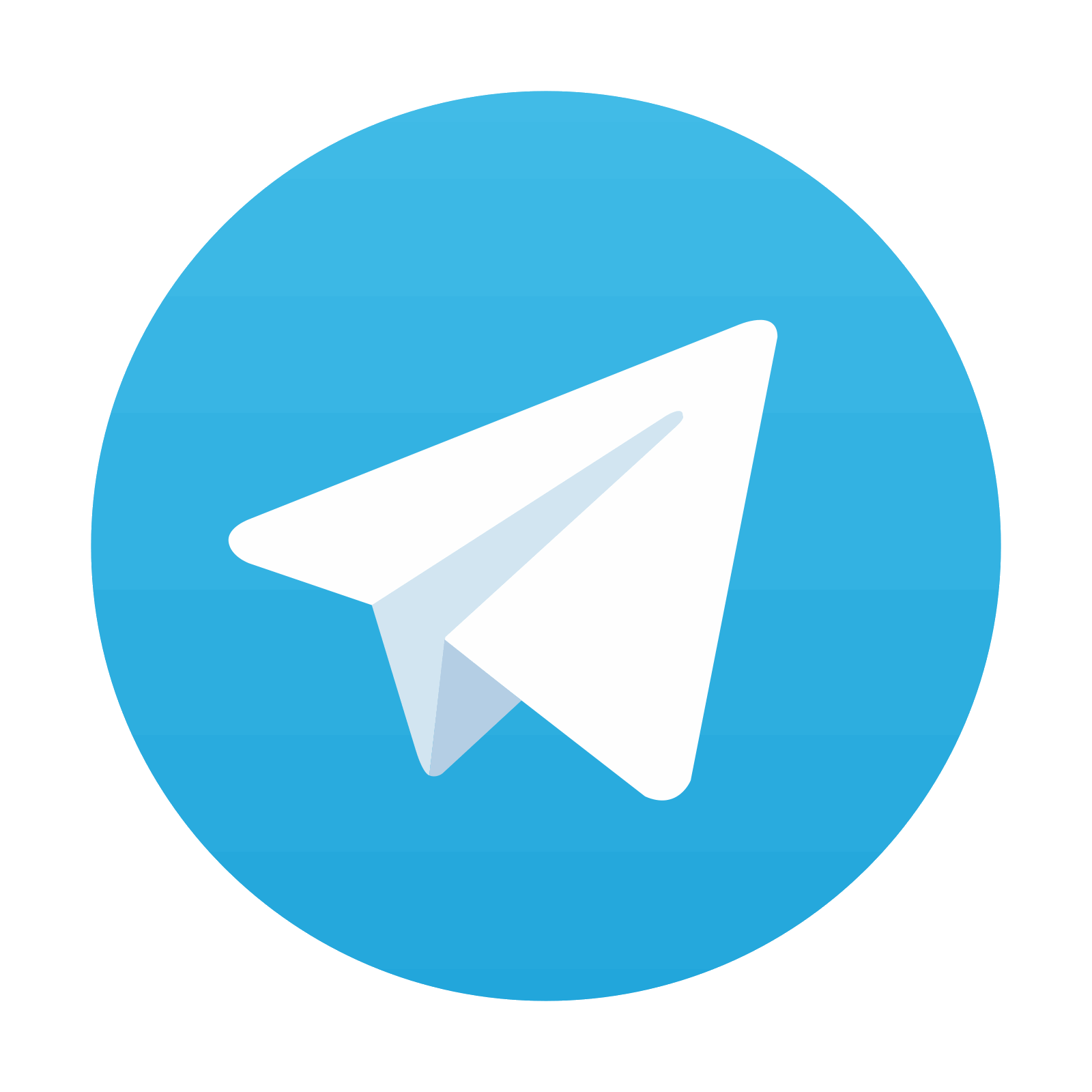
Stay updated, free articles. Join our Telegram channel
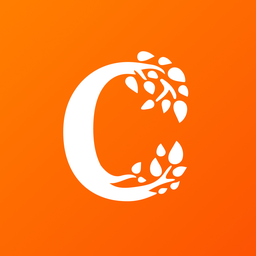
Full access? Get Clinical Tree
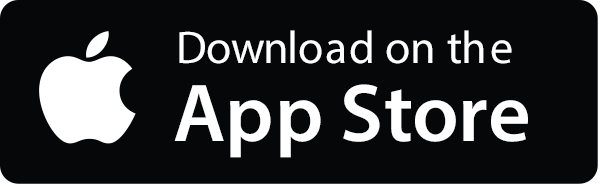
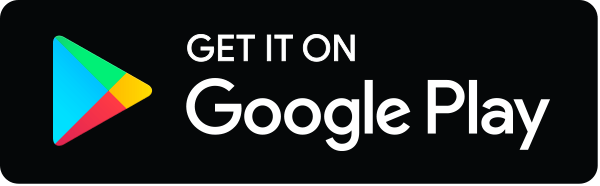