A 70-kg adult has about 100 g of sodium, 95 g of chloride, and 140 g of potassium in the body (Forbes, 1987). Distribution of these electrolytes within the various fluid compartments and body tissues is highly variable in terms of concentration and content. Sodium and chloride are the major electrolytes found predominantly in extracellular fluid, whereas potassium is retained inside the cells (Figure 34-1). The concentration of sodium ions (Na+) in the extracellular fluid, and thus in the plasma, is maintained within narrow limits at approximately 145 mmol/L. Its concentration in the intracellular fluid is low, about 12 mmol/L. Distribution of chloride ions (Cl–) generally follows that of Na+, with an extracellular concentration of about 110 mmol/L and an intracellular concentration of about 2 mmol/L. About one third of the body’s sodium is sequestered as part of the integral structure of skeleton and therefore is not available for exchange with the fluid compartments. The concentration of potassium ions (K+) is 150 mmol/L inside the cells and 4 to 5 mmol/L in the extracellular fluid. The largest fraction of body potassium, about 60% to 70% of the total, is located in the skeletal muscles, which make up about 40% of body weight. Plasma membranes surrounding cells separate the body fluid compartment into an extracellular and an intracellular component. Movement of ions across cell membranes can occur by passive diffusion along a concentration or electrical gradient through ion channels or by an energy-yielding process of active transport of ions against these gradients. The selective permeability of the cell membranes prevents the movement of proteins and phosphates out of the cells. High concentrations of these intracellular organic anions require neutralization of the excess negative charges by cations, of which K+ is the most important (see Figure 34-1). The intracellular accumulation of K+ in exchange for Na+, against their concentration gradients, is achieved by the Na+,K+-pump of the plasma membrane. This magnesium ion–dependent, Na+– and K+-activated ATPase (Na+,K+-ATPase) is the molecular basis of the Na+,K+-pump. The Na+,K+-ATPase belongs to the P-type ATPases, which are characterized by the transient phosphorylation of the ATPase protein during the transport cycle. Structurally, the functional unit of this enzyme is a heterodimer of two subunit proteins, the α-subunit and a smaller β-subunit. Other smaller membrane peptides, such as the γ-subunit, have been found to be closely associated with Na+,K+-ATPase, but their presence does not seem to affect the expression of the functional enzyme (Horisberger and Doucet, 2007). Several isoforms of both the α- and β-subunits have been identified in a variety of tissues; the relative proportion of each subunit isoform varies among tissues, and the isoforms are tissue-specific. At present, four different genes of α-subunits and three of β-subunits have been identified in mammalian Na+,K+-ATPases. Aside from their kinetic characteristics and tissue distribution, little is known regarding their differential physiological importance. The larger α-subunit (∼120 kDa) spans the membrane and has two large cytoplasmic domains. It binds ATP and both Na+ and K+ and contains the phosphorylation site. It is responsible for transport of Na+ and K+. The smaller β-subunit (∼50 kDa), with a large extracellular domain, is a glycoprotein that also spans the membrane. It is an essential component of the functional Na+,K+-ATPase. Affinity of the α-subunit for Na+ and K+ appears to be influenced by the particular β-subunit. As illustrated in Figure 34-2, the α-subunit binds ATP and three Na+ from the cytoplasm. ATP activates the catalytic site of the transporter, which then cleaves the ATP and phosphorylates the aspartate residue on the α-subunit. A conformational change in the transport protein exposes the three Na+ to the outside. The three Na+ dissociate from the enzyme and are released into the extracellular fluid. The transporter is now available for binding two extracellular K+. Binding of K+ results in dephosphorylation of the α-subunit. The transport protein returns to its original conformation, and two K+ are released into the cytosol in the process. The transport protein is ready for another cycle of transport. The concentrations of the three major ions in body fluids are controlled within narrow limits in order for the body to function properly. The volume of the extracellular fluid compartment (interstitial fluid and plasma) is determined primarily by the total amount of osmotic particles present. Because of its high concentration, Na+, together with its accompanying anions Cl– and HCO3–, is the major determinant of osmolarity of plasma and extracellular fluid. The osmolarity of plasma and body fluids, at 290 mmol/L, can be estimated by doubling its Na+ concentration of 145 mmol/L. Any change in plasma Na+ concentration will alter plasma osmolarity, or tonicity, and trigger physiological regulatory mechanisms to adjust water intake and water excretion to restore the osmolarity toward normal. Because the volume of extracellular fluid depends on the amount of Na+ present in the extracellular space, regulation of plasma volume and extracellular fluid volume involves the regulation of ingestion and excretion of sodium (Hall, 2011d). Therefore, in the control of body fluid balance, sodium and water are the two primary variables, and balance can be achieved by adjustments of their ingestion and excretion. Absorption of dietary sodium in the small intestine and reabsorption of filtered sodium from the glomerular filtrate in the kidneys back into the circulation is important in many nutrient transport processes, for example, the absorption of chloride, amino acids, glucose, galactose, and water. Transepithelial transport of Na+ involves movement across two membranes: the apical membrane facing the lumen of the intestine or the renal tubules, and the basolateral membrane, which faces the interstitial space. These two membranes have different transport properties. The apical membrane contains very few if any Na+,K+-ATPase transporters, whereas the basolateral membrane contains a large amount of Na+,K+-ATPase. Active extrusion of Na+ across the basolateral membrane by the Na+,K+-ATPase reduces the concentration of intracellular Na+ and provides an electrochemical potential gradient for the movement of Na+ from the lumen into the cytosol to replace the Na+ that is extruded. This transepithelial transport of Na+ creates an electrochemical potential difference for Na+ across the epithelial cells so that the epithelial cells are polarized, with the lumen slightly electronegative with respect to the interstitial fluid on the basal side of the epithelial cells (Hall, 2011e). Entry of Na+ down an electrical gradient across the apical membrane is either through the voltage-dependent Na+ channels or by carrier-mediated transport (facilitated diffusion) in which other solutes are cotransported in the same direction or countertransported in the opposite direction. Absorption of Na+ causes either the entry of an equimolar amount of Cl– in the same direction as Na+ movement or the secretion of an equimolar amount of K+ or H+ in the opposite direction in exchange for Na+ (Figure 34-3). The absorption of Cl– is either through the paracellular spaces (junctions between the apical borders of adjacent epithelial cells) or across the epithelial cells via the apical and basal membranes. Uptake of Na+ and Cl– across epithelial cells increases the intracellular concentrations of these two ions and provides an osmotic gradient for the absorption of water. A large proportion of water uptake occurs by the paracellular route, and only a small proportion of water flux is through the epithelial cells. Some of the carrier proteins in the apical membrane, such as the sodium/glucose cotransporter 1 (SGLT1) and a number of the amino acid transport systems, have receptor sites for binding both Na+ and a monosaccharide, or both Na+ and an amino acid (see Figure 34-3). Because Na+ is coupled to either a monosaccharide or an amino acid on the carrier protein, entry of Na+ across the apical membrane also brings in a monosaccharide or an amino acid. This process of cotransport of these organic solutes is frequently referred to as secondary active transport because the energy used to establish the Na+ gradient allows cotransport of these solutes to occur against their concentration gradients. The increase in concentrations of these organic solutes inside the epithelial cells then allows the diffusion, usually facilitated by another carrier, of these nutrients across the basolateral membrane into the interstitial fluid. The high concentration gradient of K+ between the intracellular and extracellular fluid is important for maintaining the normal resting membrane potential across cell membranes and for excitability of nerves and muscles. It plays a crucial role in the triggering of action potentials that initiate nerve impulse transmission and muscle contraction. Changes in the concentration of K+ in the plasma alter this gradient and will adversely affect the aforementioned functions (Rodriguez-Soriano, 1995). For instance, in hyperkalemia, when the concentration of K+ in the plasma exceeds 5.5 mmol/L, the membrane depolarizes, causing muscle weakness, flaccid paralysis, and cardiac dysrhythmias. Cardiac dysrhythmias associated with hyperkalemia range from sinus bradycardia to ventricular tachycardia, ventricular fibrillation, and ultimately cardiac arrest (asystole) when a plasma concentration of 8 mmol/L is reached. Because of the adverse effects of hyperkalemia on the heart, hyperkalemia constitutes a medical emergency. In contrast, in hypokalemia, when the concentration of K+ in the plasma is less than 3.5 mmol/L, the membrane hyperpolarizes, and this can interfere with the normal functioning of nerves and muscles, resulting in muscle weakness and decreased smooth muscle contractility. Hypokalemia is also a risk factor for atrial and ventricular dysrhythmias (He and MacGregor, 2008). Severe hypokalemia can lead to paralysis, metabolic alkalosis, and death. Therefore physiological regulatory mechanisms are present to regulate the concentration of K+ in the plasma within narrow limits. Activators of enzyme-catalyzed reactions are frequently metal ions such as magnesium (Mg2+), zinc (Zn2+), manganese (Mn2+), and calcium (Ca2+); only a limited number of enzymes require the presence of Na+, K+, or Cl–. The most common and most widely distributed enzyme in the cell membrane is the Na+,K+-ATPase, the activation of which requires the presence of Na+ and K+. Enzymes that require the presence of Cl– for activation include the angiotensin-converting enzyme (ACE) that catalyzes the conversion of angiotensin 1 to angiotensin 2 (Bunning and Riordan, 1987). Although the presence of Mg2+ is required for activation of a number of enzymes by K+ in mammalian tissues, K+ by itself is important for the activity of pyruvate kinase (Larsen et al., 1994). Obligatory loss of fluids through skin, feces, and urine inevitably causes loss of sodium and chloride (see Chapter 35). Minimum obligatory loss of sodium in the absence of profuse sweating and gastrointestinal and renal diseases has been estimated to be approximately 0.04 to 0.185 g/day, which consists of 0.005 to 0.035 g/day in urine, 0.01 to 0.125 g/day in feces, and dermal losses of 0.025 g/day (Dahl, 1958). Studies over a 12-day period have shown that sweat and fecal excretion accounted for only 2% to 5% of total sodium excretion in adults who consume an average intake of salt; the remainder of the salt consumed was excreted in the urine (Sanchez-Castillo et al., 1987b). However, loss of sodium can increase greatly under certain circumstances, such as diarrhea, diabetes, and profuse sweating during strenuous physical activity in hot weather. Under most but not all circumstances, loss of sodium is accompanied by a similar molar loss of chloride. As with sodium and chloride, obligatory loss of fluid also causes loss of potassium. In a healthy adult, potassium loss via these routes is less than 0.8 g/day, with less than 0.4 g via fecal loss, 0.2 to 0.4 g via urinary loss, and negligible amounts from sweat (National Research Council, 1989). Dietary sodium is consumed mainly as sodium chloride, with small amounts as sodium bicarbonate, sodium glutamate, and sodium citrate. These sodium salts are low in fresh vegetables and fresh fruits but high in cured meat products, processed foods, and canned vegetables. Studies in a British population found that only 10% of sodium intake came from natural foods, 15% from table salt added during cooking and at the table, and 75% from salts added during manufacturing and processing (Sanchez-Castillo et al., 1987a). The amount of chloride consumed parallels the amount of sodium, as dietary chloride is consumed almost exclusively as a salt of sodium. Americans consume between 1.8 and 5 g/day of sodium or between 4.8 and 13 g/day of sodium chloride (National Research Council, 1989). This wide range of reported intakes is due to the different methods of assessment and to the large variability of discretionary salt intake. Individuals consuming a diet high in processed foods have high salt intakes. In Japan, where consumption of salt-preserved fish and the use of salt for seasoning are customary, salt intake is high, ranging from 14 to 20 g/day (Kono et al., 1983). Conversely, salt consumption estimated from urinary sodium excretion of the Yanomami Indians in Brazil is very low, 0.053 g/day of sodium chloride (Mancilha-Carvalho and Souza e Silva, 2003). Vegetarians typically consume an average of 0.8 g/day of sodium chloride. Individuals with low or very low sodium intakes do not normally exhibit chronic deficiencies because of the efficiency of the body’s mechanism of salt conservation. Daily minimum requirements of sodium and chloride in the adult can be estimated from the amount needed to replace obligatory losses. This amounts to not more than 0.18 g/day of sodium when substantial sweating does not occur (Institute of Medicine [IOM], 2004). The IOM does not establish an Estimated Average Requirement (EAR), and hence Recommended Dietary Allowance (RDA), for sodium because of inadequate data from dose–response studies. Instead Adequate Intakes (AIs) through the life span are provided. Development of hypertension appears to be associated with salt intake. Several trials have demonstrated that dietary sodium intake close to the AI (e.g., 1.2 g/day) was associated with lower blood pressure, compared to higher intakes (e.g., 2.3 g/day) (Johnson et al., 2001; Sacks et al., 2001; MacGregor et al., 1989). Based on these studies, the IOM (2004) has set a Tolerable Upper Intake Level (UL) for adults of 2.3 g (100 mmol)/day of sodium and 3.6 g (100 mmol)/day of chloride. The ULs for young children are somewhat lower. Potassium is widely distributed in foods, especially in vegetables and fruits. Intakes of potassium vary widely, depending on dietary habits. Based on the NHANES III data, the median intake of potassium in the United States is about 3.3 g/day for men and 2.2 g/day for women (IOM, 2004). These values do not include the use of salt substitutes or reduced-sodium salts. Salt substitutes contain from 0.4 to 2.8 g potassium per teaspoon as potassium chloride. Because of the growing evidence of the beneficial effects of potassium in protecting against cardiovascular diseases and reducing cardiovascular disease mortality, concern of possible underconsumption of potassium has led to recommendations for increased intake of dietary fruits and vegetables (He and MacGregor, 2008). To replace the obligatory loss of potassium, an adult should consume not less than 0.8 g/day of potassium. The IOM (2004) did not set an EAR or RDA for potassium because of insufficient dose–response data to establish an EAR. The adult AI for potassium was set at 4.7 g/day based upon the dietary level that would blunt the severe salt sensitivity prevalent in black men (Morris et al., 1999). This level of AI for potassium intake is also associated with a lower blood pressure, lower risk of kidney stones, and decreased bone loss. No increment was added to the AI for pregnant women, but the AI for lactating women was increased to 5.1 g/day to allow for the potassium content in human milk secreted during the first 6 months of lactation. Based on the NHANES III data, the percentage of adults who consume an amount of potassium that is equal to or greater than the AI is only 10% for men and less than 1% for women in the United States (IOM, 2004). A diet rich in fruits and vegetables is necessary to obtain the AI for potassium from natural foods. There is no evidence that a high level of potassium from foods has adverse effects, so a UL for potassium was not set by the IOM (2004). However, in individuals with impaired urinary excretion of potassium, chronic consumption of a high level of potassium can result in hyperkalemia. Supplemental potassium should be used only under medical supervision in this case. These electrolytes are filtered freely from the glomerular capillaries across the glomerular membrane into the Bowman’s space of the nephrons (functional units of the kidneys) so that their concentrations in the glomerular filtrate are similar to those in the plasma. As the filtrate flows along the renal tubules, reabsorption of Na+, Cl–, and K+ and secretion of K+ by renal tubular cells alter the concentrations of these electrolytes in the urine. Figure 34-4 illustrates the basic renal processing of Na+, Cl–, and K+. Normally 95% to 98% of the filtered load of these ions and water are reabsorbed by the proximal tubules, the thick ascending limbs of the loop of Henle, and the distal convoluted tubules, irrespective of intakes. Reabsorption of the remaining 2% to 5% of the filtered Na+, Cl–, and water, and the secretion of K+ in the cortical collecting tubules are variable and depend greatly on the needs of the individual. When intake of sodium is high, reabsorption of Na+ from the cortical collecting tubule is low so that the excess Na+ is excreted in the urine. However, when there is a sodium deficit due to low intake or excessive loss via the skin or the gastrointestinal tract, much of the Na+ in the filtrate is reabsorbed, resulting in very low Na+ concentration in the urine. In contrast to Na+, K+ is secreted by the tubular cells of the cortical collecting tubules (Rodriguez-Soriano, 1995). In the absence of disease, changes in K+ excretion are not due to changes in reabsorption of K+ in the proximal tubules but to changes in K+ secretion by the cortical collecting tubules. During potassium depletion, most of the filtered K+ is reabsorbed, and virtually no K+ is excreted. The small amount of K+ excreted comes from the filtered K+ that escaped reabsorption. However, when potassium intake is high, secretion of K+ is increased, thereby eliminating the excess potassium. A normal Western diet contains about 2 to 3 g/day of potassium and requires secretion of K+ in order to maintain potassium balance. No important receptors capable of detecting the amount of sodium in the body have been identified. However, because Na+ is the main determinant of extracellular fluid volume, physiological mechanisms that control the volume of extracellular fluid effectively maintain a balance for sodium and chloride (Hall, 2011c). Changes in extracellular fluid volume lead to corresponding changes in the effective circulating volume and affect the “fullness” or “pressure” in the circulation. This changes cardiac filling pressure, cardiac output, and arterial pressure. Volume or pressure sensors (baroreceptors) that detect these changes are located throughout the vascular system. These baroreceptors send either excitatory or inhibitory signals to the central nervous system and the endocrine glands to effect appropriate responses by the kidneys to match the amount of sodium ingested. Three major mechanisms participate in the regulation of sodium balance: (1) vascular pressure receptors and their efferent renal sympathetic and arginine vasopressin (AVP) pathways, (2) the renin–angiotensin–aldosterone system, and (3) natriuretic peptides. Two of these mechanisms, vascular pressure receptors and their corresponding efferent pathways, and the renin–angiotensin–aldosterone system, respond effectively to hypovolemia by conservation of body sodium and water, whereas the natriuretic peptides are effective in hypervolemia when excess sodium and water are excreted in the urine (Hall, 2011b, 2011c, 2011d).
Sodium, Chloride, and Potassium
Functions and Distribution of Sodium, Chloride, and Potassium
Distribution in the Body
Properties of Plasma Membranes
Sodium–Potassium Pump
Importance of Sodium
A Major Determinant of Osmolarity and Extracellular Fluid Volume
Role of the Electrochemical Sodium Gradient in Nutrient Transport Processes
Importance of Potassium
Other Functions of Electrolytes
Interactions with Macroions
Activation of Enzymes
Sodium, Chloride, and Potassium Balance
Loss of Sodium, Chloride, and Potassium
Intake of Sodium and Chloride
Dietary Intake
Recommended Intake
Intake of Potassium
Dietary Intake
Recommended Intake
Regulation of Sodium, Chloride, and Potassium Balance
Renal Excretion of Sodium, Chloride, and Potassium
Control of Renal Excretion of Sodium and Chloride
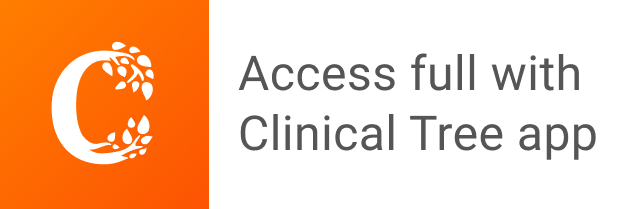