Fig. 13.1
Schematic illustration of IS-FET
Recently, FET biosensors are being studied and developed to apply for clinical diagnosis, drug discovery, tissue engineering, and so on. Since FET biosensors can detect molecular recognition events accompanied by charge density changes without labeled materials and be easily arrayed by the use of the conventional semiconductor process which enables to measure multi-samples, the platform based on FET chips is suitable for a simple and cost-effective system for chip-based diagnosis. The downsizing of a system is significant for personalized medicine at home. Moreover, the electrical signals based on FET devices result in direct and quantitative analyses of bio-samples. One positive or negative charge of an ion or ionic molecule interacts electrostatically with one electron charge in a semiconductor device. Therefore, ion behaviors based on biological phenomena can be directly detected using semiconductor devices. Most of biological phenomena in vivo are closely related to electrical behaviors of charged media, for example, DNA molecules with negative charges based on phosphate groups, ions (potassium ions, sodium ions, and so on) through ion channels at the cell membrane keeping homeostasis in a body, and so on.
13.2 Concept of Semiconductor-Based Biosensing Devices
13.2.1 Ion-Sensitive Field-Effect Transistor (IS-FET)
As a reason of a bad tooth, three elements of Streptococcus mutans, “quality of tooth,” and “saccharinity” affect on a bad tooth in the course of time. Streptococcus mutans induces acidification by dissolving saccharides in food and drink. As a result, acidification of dental plaque is in progress on a tooth. That is to say, the enamel of the tooth begins to dissolve to less than pH 5.5 resulting in a bad tooth. Therefore, it is important to control meals considering pH variation in a mouth in order to prevent a bad tooth. Thus, pH measurement is needed even for health care in daily life and can be accomplished by engineering such as semiconductor technology.
The principle of IS-FET is based on potentiometric detection of charge density changes induced at a gate insulator/solution interface accompanied by pH variation. Hydrogen ions with positive charges at the gate insulator electrostatically interact with electrons in a silicon crystal across the thin gate insulator resulting in the V T change.
Typical drain voltage (V DS)–drain current (I D) characteristic of the FET is shown in Fig. 13.2. It is found that the FET can be operated correctly. Since the fabricated FET is a depletion type as can be seen in Fig. 13.2, the reference electrode is usually connected to the ground for the measurement of the interfacial potential between the gate insulator and solution using the circuit shown in Fig. 13.3. The pH-response characteristics of the FET with a Si3N4 gate are shown in Fig. 13.4. The time course of the interfacial potential was measured during calibration and is shown in Fig. 13.4a. The arrows indicate the timing to change the buffer solutions. The interfacial potential changed rapidly after changing the buffer solution and became stable within 1 min. The calibration curve for the Si3N4 gate FET is shown in Fig. 13.4b. The relationship between pH and the output voltage is linear in the range from pH 1.68 to pH 9.18 with a correlation coefficient of 0.9999. The slope of the calibration curve was 57.52 mV/pH, which is close to the theoretical slope at 25 °C. On the basis of these results, the operation of the Si3N4 gate FET was considered to be stable and no leakage through the gate insulator and no defect of the encapsulation could be observed.




Fig. 13.2
V DS–I D characteristic of IS-FET

Fig. 13.3
Measurement circuit using IS-FET

Fig. 13.4
(a) Change of output voltage for each pH and incubation time. (b) Correlation between output voltage and pH. Each output voltage for pH was averaged in the last 10 s before changing buffer solution. All of the measurements were performed at 25 °C
13.2.2 Device Structures
An insulated-gate field-effect transistor (IG-FET) is composed of electrolyte–insulator–semiconductor. The structure is utilized as a pH sensor of IS-FET. The electrical characteristics of IG-FET are the same as the ones of IS-FET shown in Sect 13.2.1.
On the other hand, an extended-gate field-effect transistor (EG-FET) is shown in Fig. 13.5. The gate electrode is separated and extended from the metal oxide semiconductor (MOS)-FET. Using the EG-FET, materials and structures of the gate electrode can be varied and gate sensing membranes can be easily arrayed by a sputtering method, and MOS-FETs are not replaced and can be reused. Various kinds of gate materials can be designed as follows:


Fig. 13.5
Extended-gate field-effect transistor for biosensing
Gold (Au) gate electrode
It is simple to immobilize biomolecules on the Au electrode, because spacer molecules with -SH (thiol group) are easily tethered. DNA or antibody molecules are immobilized by binding with spacer molecules on Au. However, the Au surface is polarized in solutions and a constant potential based on ion charges is not determined. Therefore, it is effective to tether the self-assembled monolayer (SAM) with both of the functional groups (−COOH, −NH2, and so on) and -SH group at each end, when the Au gate electrode is utilized as the sensing surface of the EG-FET for biosensing, because their functional groups are bonded with various biomolecules.
Indium–tin–oxide (ITO)-gate electrode
Transparent electrode is available for microscopic observation of cultured cells as if electrical monitoring is performed using a conventional culture dish. The ITO is basically oxide so that its surface is covered by hydroxyl groups in a buffer solution. The potential of the EG-FET with the ITO gate shows a near-Nernstian response to pH variation [5]. However, it is very important to control the making process of the ITO surface.
Scaffold gate electrode
Using the EG-FET, the structure of the gate itself can be varied corresponding to applications. In the field of tissue engineering, the safety and functionality of artificial organs are required to transplant to a living body actually. This is why 3D culture of cells is required for making artificial organs. In order to perform 3D culture on the electrode, a nano- or microfiber scaffold is able to be developed as a gate electrode of EG-FET. Scaffold architecture affects cell binding and spreading. Cells binding to scaffolds with microscale architectures flatten and spread as if cultured on flat surfaces, while the scaffolds with nanoscale architectures have larger surface areas to adsorb proteins, presenting many binding sites to cell membrane receptors. The adsorbed proteins may also change conformation, exposing additional cryptic binding sites [6].
Cantilever gate electrode
A biosensing technique to measure the molecular charge distribution at the cell membrane is proposed using a principle of semiconductor. A cantilever of atomic force microscopy (AFM) is utilized in order to be in contact with the cell membrane and to move the electrode at nanoscale, and a “gate lever” with a platinum tip is studied to detect charges at the cell membrane using AFM. The gate lever as an electrode is extended from the MOS-FET as one of the EG-FET. Actually, negative charges based on sialic acids and so on at the cell membrane can be detected using the gate lever-FET. As a result, the distribution of the membrane protein with some charges at the cell membrane can be mapped at nanoscale using the proposed system.
The device structure for biosensing is composed of three functional elements such as the biological target, signal transduction interface, and detection device, as shown Fig. 13.6. In developing biosensing devices, a specific biological target should be designed for application. In this case, such a detection target is found in the field of medicine, biology, pharmaceutical discovery, tissue engineering, clinical diagnosis, food safety, and so on. In order to detect biological targets selectively, a signal transduction interface should be designed between the target and detection device. The functional interfaces are formed between solid and liquid phases, which can be classified as a physically structured interface, chemically modified interface, and biologically induced interface. Lastly, detection devices should be designed as sensing principles that enable to detect mass, viscoelasticity, charges, permittivity, fluorescence, and so on. In this chapter, the semiconductor-based biosensing devices are introduced as the detection device, but various kinds of materials of semiconductors are designed such as silicon, carbon nanotube, graphene, transparent amorphous oxide, and so on.


Fig. 13.6
Device structure for biosensing
13.3 Signal Transduction Interface
13.3.1 Physically Structured Interface
The micro- and nanostructures on the gate electrode of semiconductor devices contribute to the increase of surface area for immobilization of probe molecules and amplification of signals (Fig. 13.7). Nanoparticles or nano-pillars can be designed as nanostructures, of which gold, silica, and carbon nanotubes, and so on are utilized. In particular, the detection limit of FET devices is closely related to the Debye length at the interface between the gate surface and solution. When the nano-pillar structure is made within the Debye length, longer or larger biomolecules such as DNA or protein can be detected within the Debye length. In the case of DNA sensing, particularly, extended DNA molecules can be detected parallel to the gate surface resulting in label-free DNA sequencing based on intrinsic molecular charges.


Fig. 13.7
Nanostructure-based FET
13.3.2 Chemically Synthesized Interface
Functional interfaces synthesized chemically are proposed as a monolayer or polymer membrane. A functional membrane as a monolayer sensitive to K+ at the gate insulator is prepared using an 18-crown-6 ether derivative, as shown in Fig. 13.8. First, carboxyethylsilanetriol was used as silane coupling agent. The sensor was immersed in carboxyethylsilanetriol sodium salt 25 wt% in water for 24 h, then rinsed with Milli-Q, and dried in a vacuum at 120 °C for 1 h. Second, 4-aminobenzo-18-crown-6 (AB18C6) was used as an ionophore of K+, and 1-ethyl-3-(3-dimethylaminopropyl) carbodiimide (EDC) was used as a condensation agent. The sensor was immersed in AB18C6 (1 mM) and EDC (10 mM) in N,N-dimethylformamide (DMF) for 24 h and rinsed. Finally, the chemical structure shown in Fig. 13.8 was synthesized. This monolayer does not contain a plasticizer, and the ionophore is chemically incorporated to the gate insulator, so there is no expected cytotoxicity.


Fig. 13.8
Crown ether-modified gate transistor for K+ detection
In order to investigate the electrical characteristics of FET, a semiconductor parameter analyzer was used. Measurement was performed in 2[4-(2-hydroxyethyl)-1-piperazinyl] ethanesulfonic acid (HEPES) buffer solution, and the pH of the sample solution was adjusted to 7.4 with Ca(OH)2 ( [Ca(OH)2] = 1.7 mM ). And K+ concentration was adjusted to 10−4 M, 10−3 M, 10−2 M, and 10−1 M at pH 7.4. The prepared FET was immersed in the sample solution, and then the V T change was measured. The effect of K+ concentration on the electrical signal of prepared FET was investigated. The electrical response of prepared FET to K+ concentration was 4.2 mV/decade. The slope was relatively small compared to that of the Nernstian response. This could be because the density of ionophore immobilized onto the FET gate surface is low. Further investigation toward improved sensitivity and selectivity to K+ is currently underway. The V T shift to the negative direction indicates the increase of K+ with positive charge on the crown ether-modified gate insulator. Thus, the change of K+ concentration was successfully detected using the prepared FET. The prepared device showed the electrical signals of potassium ion release through the cell membrane caused by programmed cell death “apoptosis.”
Also, measurements of DNA molecular recognition or antigen–antibody reaction require chemical immobilization of probe molecules for target molecules using spacer molecules of a monolayer or polymer membrane.
13.3.3 Biologically Induced Interface
Figure 13.9 shows a schematic illustration of a biologically induced interface. A cell A is composed as a functional interface cell and induces a signal based on a target cell B to a semiconductor device as if an interface material plays its role. A cultured cell A causes a function of cell B due to cell–cell interaction on the gate sensing surface. Here, the vascular endothelial cell and tumor cell correspond to cell A and B, respectively, for example. The noninvasive and real-time measurement of invasion of cancer cells to the vascular endothelial cell layer and basement membranes was performed using the vascular endothelial cell-based field-effect transistor (VEC-FET), which is based on potentiometric detection of molecular recognition on the gate insulator. The shift of the V T caused by charge density changes on the gate insulator can be monitored during the invasion process of tumor cells. The negative shift of the V T has been successfully detected around 4 h after addition of invasive tumor cells (HeLa cells). This result indicates that the positive charges were induced on the surface of the gate insulator as a result of degradation of a negative-charged basement membrane on the gate insulator by secretion of enzyme from tumor cells. Thus, we can design in vivo-like gate FET in order to detect and reproduce biological events in vivo such as cell–cell interaction on the gate sensing surface in vitro. The platform based on the biologically induced gate FET is suitable for an in vivo-like sensing.


Fig. 13.9
Biologically induced interface
13.4 In Vitro Cell Sensing with Semiconductor Platform [7–16]
In vitro cell sensing results in the significant information of cell functions conveniently. When artificial cells such as an induced pluripotent stem (iPS) cell are transplanted into a body after control of differentiation induction, their functions contribute to regeneration of tissues or organs. In this case, the regenerated cells are required to be safe and show good quality in a body. So, the evaluation of cellular activities is needed to check the quality of living cells before transplantation. On the other hand, the effect of drugs on living cells is being investigated including a side effect in the field of pharmaceutical discovery. In this case, dying cells, such as programmed cell death, apoptosis, are detected by cell sensing methods. Thus, in vitro cell sensing plays important roles for quality check of transplanted cells or evaluation of degradation of cells based on drug effects in the field of regenerative medicine, clinical diagnosis, drug screening, and so on. Here, some case studies of in vitro cell sensing are introduced as follows.
13.4.1 In Vitro Monitoring of Cellular Respiration Activity
Glucose-stimulated electrical activity in pancreatic β-cells was provided as evidence of cell electrical activity [17], although muscle and nerve cells were believed to be specialized cells with electrical excitability. After the investigation, numerous works on the electrophysiology of β-cells were carried out and it has been elucidated [18–20]. The patch-clamp technique makes it possible to demonstrate the channels that contribute to the generation of electrical activity. The adenosine triphosphate (ATP)-regulated K+ channels (KATP channels) provide the crucial link between metabolic and electrophysiological effects of glucose. K+ flows out of the cell through spontaneously active KATP channels at low glucose and ATP concentrations. The K+ gradients existing over the β-cell membrane result in a negative membrane potential of approximately −70 mV. Increasing glucose concentrations subsequently elevates intracellular ATP concentrations and closes KATP channels. Moreover, the generation of electrical activity is a key step in glucose-induced insulin release, and it is widely thought to represent the primary mechanism by which Ca2+ is imported into the β-cell cytosol, causing an increase in intracellular Ca2+ concentration and triggering several processes that ultimately result in insulin exocytosis [21].
Basically, the semiconductor-based biosensing device that was used in this study is IS-FET, which has been commonly used as a pH sensor [3]. The principle of IS-FET is based on the direct detection of hydrogen ion concentration based on the site-binding model [22] at the gate insulator, as also described above. On the other hand, the glucose-induced insulin secretion process includes the activation of ATP generation, which is closely related to respiration activity in the mitochondrion. The induction of respiration activity would increase hydrogen ion concentration at the cell/gate interface because of CO2 release in solutions [23]. As a result of activation of respiration, the increase of hydrogen ion concentration at the cell/gate interface will be directly detected as pH variation using IS-FET. In this section, we introduce the real-time, label-free, and noninvasive monitoring of electrical activity of rat pancreatic β-cells using a cell-based FET, focusing on the correlation between the respiration activity accompanied by the insulin secretion process due to glucose induction and electrical activity of pancreatic β cells.
Rat pancreatic β-cells (RIN-5 F) were used for the electrical detection of glucose response using the FETs in the present study. The β-cells were introduced to the sensing areas of the FET chip in a RPMI1640 culture medium, pH 7.4 (Invitrogen), including 2 mg/ml glucose supplemented with 10 % fetal bovine serum (FBS; JRH Biosciences) and 1 % penicillin/streptomycin (Invitrogen) at 37 °C in an atmosphere of 5.0 % CO2 for 3 days after being maintained on a culture dish with the controlled RPMI1640 medium at 37 °C in an atmosphere of 5.0 % CO2 for 1 week. In the electrical measurements, 400 μl of the controlled medium without glucose was placed in the FET chamber, and 10 mg/ml glucose was added to the culture medium at 10 μl. Image analyses were carried out using an optical microscope in parallel with electrical detection.
Figure 13.10 shows the concept of the cell-based FET. The diagram for the measurement of electrical signals is shown in Fig. 13.10a. The pancreatic β-cells were cultured on the gate surface of the FET, which is composed of the Ta2O5/Si3N4/SiO2 layers. The Ta2O5 gate surface was not chemically modified with functional molecules. The potential of a measurement solution is controlled using a Ag/AgCl reference electrode with saturated KCl solution. Two types of FETs were prepared in the present study; one is the cell-based FET with pancreatic β-cells cultured on the gate surface, and the other is the reference FET without cells (Fig. 13.10b). Using these FETs, differential measurements were performed in order to eliminate the common background noises such as temperature changes and changes in ion concentration.


Fig. 13.10
Schematic illustration of cell monitoring. (a) Structure of cell-based FET. (b) Differential measurement between reference and cell-based FETs
Figure 13.11 shows the shift of the surface potential of gate sensing area after introduction of glucose at a concentration of 10 mg/ml using the pancreatic β-cell-based FET. The β-cells aggregated on the Ta2O5 gate surface in the culture medium, because it was difficult for β-cells to adhere to the nonmodified gate but easy for them to aggregate like a spheroid. As shown in Fig. 13.11, the surface potential of the β-cell-based FET shifted gradually in the positive direction at about 8.5 mV for about 3 h. The average of surface potential changes due to the introduction of glucose was 10.3 mV for about 3 h using five-cell-based FETs. On the other hand, almost no surface potential changes of the reference FET was found, although a smaller shift was detected owing to the background noises such as temperature changes when glucose was injected to the gate. The electrical signal of the β-cell-based FET indicates the increase in positive charges or the decrease in negative charges based on ions or charged molecules through β-cells at the β-cell/gate interface following the introduction of glucose into the β-cell-based FET. About five β-cells were cultured on the gate sensing area, of which the gate width was 10 μm and the gate length was 340 μm. The surface potential changes were based on the charge density changes of the glucose response of about five β-cells. The uptake of glucose into β-cells causes the increase in ATP/ADP concentration, the depolarization of KATP channel, the influx of Ca2+ through ion channel, and the exocytosis of insulin and C-peptide together with Zn2+ to form microcrystals within secretory granules [24]. In this study, insulin and C-peptide secretion has been detected by the use of enzyme-linked immunosorbent assay (ELISA) method based on the reaction with an anti-insulin monoclonal antibody, of which the concentration was about 32 ng/ml at 2 h after glucose addition. The resulting molecular and ion charges at the β-cell/gate interface induced the surface potential changes of the cell-based FET that prove the electrical activity of pancreatic β-cells. Furthermore, the positive shift of surface potential can be assumed to indicate the increase in the concentration of hydrogen ions at the β-cell/gate interface, because the nonmodified FET device with the Ta2O5 gate insulator is basically more sensitive to hydrogen ion concentration than those of other ions. This is why we assume that the respiration of β-cells would have been activated during the insulin secretion process following glucose addition. After the increase of electrical signal, the surface potential of β-cell-based FET decreased gradually because of diffusion of hydrogen ions from the cell/gate interface to the bulk solution accompanied by degradation of respiration activity. The insulin secretion process would include the respiration activity of β-cells so that the electrical monitoring by the use of the β-cell-based FET might demonstrate the reduction and exhaustion of glucose-induced insulin secretion.


Fig. 13.11
Electrical monitoring of pancreatic β-cell activity
The pH variation at the β-cell/gate interface determined on the basis of respiration activity can be estimated from the electrical signals of the cell-based FET. Basically, the FET chip utilized in this measurement showed the change of gate voltage 51.4 mV/pH for pH variation. The average change in surface potentials after the addition of glucose was about 10.3 mV with a standard error of ±2.2 mV for five-cell-based FETs. Therefore, the respiration activity triggered by glucose caused the change of about pH 0.2 at the interface between the pancreatic β-cells and the gate surface. Strictly, the shift of pH from 7.4 to 7.2 was detected at the β-cell/gate interface by the cell-based FETs. The amount of eliminated CO2 was calculated to be about 1.2 × 10−8 M on the basis of principle that changes in hydrogen ion concentration corresponds to pH variation, according to the equilibrium of CO2 in solutions. In the calculation, O2 consumption, ATP synthesis, and so on in the citric acid cycle inside the mitochondrion could be estimated from the amount of eliminated CO2, which was determined from the electrical signals of the cell-based FET. However, the effect of a buffer solution on pH and the diffusion of CO2 at the β-cell/gate interface have to be considered in order to estimate accurately the consumption and the generation based on the respiration activity. Also, the electrical signals of the β-cell-based FET seem to include the effect of ion or molecular charges in the insulin secretion process other than the pH variation based on respiration activity. In a previous work [13], the noninvasive monitoring of transporter function was shown using oocyte-based FETs. The size of an oocyte was about 1 mm and larger than that of a somatic cell (about 10 μm). Therefore, the amplification of electrical signals based on the transporter function was expected by the use of the oocyte-based FETs. However, the electrical detection of β-cell activity obtained in this study has demonstrated the possibility of detection of the somatic cell function using β-cell-based FETs.
13.4.2 Evaluation of In Vitro Fertilization (IVF)-Based Embryo with Semiconductor Principle
Recently, assisted reproductive technology (ART) has been expected to be one of the therapeutic methods of sterility. Engineers other than obstetricians have been required for assured success of ART programs. For in vitro fertilization (IVF) of one of ART programs, how to evaluate embryo quality and select embryo in condition are significant. Morphological evaluation has been widely used to rank embryo quality because microscopic analysis is noninvasive and useful in predicting pregnancy rates [25, 26]. However, the standard of classification for embryo quality seems to be ambiguous among operators because it is a subjective method. Moreover, elective single-embryo transfer (eSET) will be recommended in the future in order to prevent multiple pregnancies [27]. Therefore, the novel principle to evaluate the quality of a single embryo quantitatively and noninvasively in a real-time manner is being required for practical use in ART.
In our previous work, the respiration activity of fertilized ova of a sea urchin was monitored noninvasively, quantitatively, and continuously as the change of pH by the use of the principle of semiconductor-based IS-FET [28]. The detection principle of IS-FET is based on the potentiometric detection of charge density changes at the gate insulator and is applied for a biosensing circuit [29]. Since the gate insulator usually consists of Si3N4 or Ta2O5 with a hydroxyl group at the surface in solutions, furthermore, the IS-FET is sensitive to the concentration of hydrogen ion with a positive charge and should be utilized as the pH sensor. Therefore, pH variation based on the respiration activity of an embryo will be monitored quantitatively and noninvasively in a real-time manner using a single-embryo-coupled gate FET for eSET (eSET-FET), because pH at the interface between an embryo and the gate membrane of FET will change sensitively according to the dissolving of carbon dioxide into a medium generated by the metabolism and respiration activity in an embryo. Thus, the platform based on eSET-FET sensor will be valuable for the development of an evaluation system to select a single embryo with good quality for eSET in the future.
On the other hand, oxygen consumption has been considered to be the parameter that provides the best indication of overall metabolic activity of a single embryo [30–34], although embryo metabolism has previously been assessed by measurement of nutrient consumption, such as glucose, pyruvate, and amino acids [35–38]. As one of the detection methods for the evaluation of embryo quality, the electrochemical system is being developed. Shiku et al. reported previously the detection concept of oxygen consumption based on the respiration activity of an embryo [39]. In this method, the oxygen reduction current was detected near the surface of a single embryo using the cyclic voltammetry technique. However, this method is unsuitable for real-time measurement for a long term such as cleavage of mammalian embryo.
In this case study, we introduce a semiconductor-based embryo sensing device for eSET (eSET-FET) to monitor single mouse embryo activity based on cellular respiration in a real-time, quantitative, and noninvasive manner. Additionally, we report to have developed the simultaneous analysis system composed of microscopic observation and electrical measurement of eSET-FET under the adequate embryo culture condition in an incubator.
Insulated gate FETs as semiconductor devices were fabricated using the standard integrated circuit technology except for deposition of the gate electrode. The N-channel depletion mode FET was designed in a 2 mm × 5 mm chip (ISFETCOM Co. Ltd.). The gate insulator was composed of Ta2O5, Si3N4, and SiO2 layers, of which each thickness was 50, 100, and 100 nm, respectively. The gate surface contacting directly with the embryo and culture medium was Ta2O5 layer, of which the safety was confirmed by estimating the rate of transplantation of cultured embryos on the surface to recipient mice. The fabricated FET chip was mounted on a flexible polyimide film with patterned copper electrodes and bonded. The FET chip was encapsulated with a glass ring except for the sensing area and was utilized in a culture medium as the eSET-FET sensor. The eSET-FET sensor was immersed in a culture medium (KSOM; Arc Resources JAPAN) with a platinum Pt reference electrode. Polydimethylsiloxane (PDMS) was utilized for the mounting of a glass ring and Pt electrode on the device because of nontoxicity for an embryo culture. A single mouse embryo was put on one gate sensing area of the eSET-FET (Fig. 13.12a). The embryologist put a single mouse embryo on a gate area by using a micropipet.


Fig. 13.12
Measurement device for embryo monitoring. (a) Photograph of a single mouse embryo on the gate of eSET-FET sensor. (b) Simultaneous embryo analysis system. Electrical measurement using the eSET-FET sensor can be continuously performed in various intervals of second or minute to ~ a few weeks or more in the incubator controlled at 37 °C and 5 % CO2. The upright microscopy was set up in the incubator. (c) Sensing structure. A droplet of 20 μl was covered by mineral oil so that a long-term embryo culture can be conducted. Mineral oil is nontoxic and has the permeability of gases such as CO2 and O2
A mammalian embryo is very sensitive to external environments such as the ion strength of culture medium, temperature, and so on. This is why the total system for evaluation of embryo based on IVF should be prepared appropriately. Figure 13.12b shows the simultaneous analysis system of embryo activity by microscopic observation and electrical measurement using the eSET-FET sensor in the incubator. Using the simultaneous analysis system, the subjective and objective evaluation of an embryo under the adequate condition of an embryo culture can be performed in a real-time and noninvasive manner. Moreover, the sensing area was covered by 20 μl of droplet culture medium, where a single mouse embryo was kept on the gate of the semiconductor, as shown in Fig. 13.12c. The mineral oil of 1 ml was introduced onto a dropped culture medium of 20 μl to prevent drying. The droplet culture is useful to control the position of a single embryo on the gate and detect ion concentration change due to low volume.
The electrical characteristics of the eSET-FET sensor such as the gate voltage (V G)–drain current (I D) characteristics and the surface potential at the gate surface were measured in a culture medium using a semiconductor parameter analyzer (B1500A, Agilent) and a custom-made potentiometric analyzer (Radiance Ware Inc.), respectively. As the basic electrical characteristic, the threshold voltage shift ΔV T was determined after an embryo was placed on the gate. The ΔV T was defined as a difference of the V G–I D characteristics at a constant drain current of 700 μA. The time course of the surface potential at the gate surface was monitored using a circuit with which the potential change at the interface between an aqueous solution and the gate insulator can be read out directly at a constant drain current. In the present study, the gate voltage and the drain current were set to be 1 V and 700 μA, respectively.
All the nutrient media to be used for IVF were prepared on the day before experiments, and a balanced gas in droplet was controlled through overnight under the adequate condition (5 % CO2 and 37 °C). Male ICR mice (9–10 weeks old; Charles River JAPAN Inc.) were sacrificed by cervical dislocation. Sperms collected from caudal epididymides of ICR male mouse were incubated for 1.5 h at 5 % CO2 and 37 °C. In this case, sperms of 2 × 105 cells/ml were seeded in HTF (human tubal fluid; Arc Resources JAPAN) droplet of 200 μl and subsequently cultured again in fresh HTF covered by mineral oil (Mineral Oil Light; REPROLINE) at 5 % CO2 and 37 °C. Female B6D2F1 mice (9–10 weeks old; Charles River JAPAN Inc.) were kept for 48 h from intraperitoneal injection of 5 IU PMSG (pregnant mare’s serum gonadotropin; ASKA Pharmaceutical JAPAN) and subsequently sacrificed by cervical dislocation after 15–16 h from intraperitoneal injection of 10 IU hCG (human chorionic gonadotropin; ASKA pharmaceutical JAPAN) for ovarian hyperstimulation. Oviducts were excised and drew to take out ova with a needle. The ova were introduced into HTF droplet with sperm for IVF. After about 5 h from insemination, treated ova were washed and moved from the fertilization medium to KSOM (potassium simplex optimized medium; Arc Resources JAPAN) droplet for culture. After the release of a second polar body from an ovum was confirmed, the embryo was set up on a gate sensing surface of the eSET-FET sensor, which is then used to measure the embryo in the incubator system with microscopy. The rate of blastocyst hatching and average of total cells in single embryo at the blastocyst-hatching stage were investigated as shown in Table 13.1. Each counting was conducted at 90 h after measurements (IVF). Total cells were counted by the use of fluorescent dye (DAPI) and confocal laser scanning microscopy. Moreover, the transplantation of embryo to recipient mice cultured on the gate of semiconductor and the culture dish was conducted in order to confirm safety of an embryo culture on the eSET-FET sensor. Actually, the blastocysts developed normally on gate during measurement were used for implantation to recipient mice. We got the Institutional Review Board approval for this study. This study was overseen by the Animal Care and Use Committee in the University of Tokyo.
Table 13.1
Rate of blastocyst hatching and average number of cells in a single mouse embryo at blastocyst-hatching stage for embryos cultured on the gate of semiconductor and the culture dish
Gate of semiconductor | Culture dish | |
---|---|---|
Rate of blastocyst hatching (%) | 88.1 | 96.8 |
Average number of cells in an embryo (%) | 71.5 | 72.4 |
It is very important to confirm firstly the safety of an embryo culture on the semiconductor devices like on a conventional culture dish. Actually, the mouse embryo after IVF was normally cultured on the gate until the blastocyst-hatching stage. Table 13.1 shows the rate of blastocyst hatching and the average of total cells in an embryo at the blastocyst-hatching stage for embryos cultured on the gate or the culture dish as control, respectively. The rates of blastocyst hatching were around 90 % for both conditions. The average of total cells for embryos cultured on the gate was also similar with that for embryos cultured on the culture dish. The culture of embryo on the gate was found to be of no difference with the one on the culture dish. Furthermore, the safety of an embryo culture on the gate was investigated by transplantation to the recipient mouse (Table 13.2). The rates of offspring were compared for IVF embryo transfer (ET) of embryos cultured on the gate or culture dish, respectively. Even embryos cultured on the gate of eSET-FET sensor obtained about the same offspring rate for IVF-ET with embryos cultured on the conventional dish. These data mean that the semiconductor device itself never show toxicity for mouse embryo under the culture condition. Thus, the embryo sensing by the use of the eSET-FET sensor was confirmed to be safe for the evaluation of embryo quality after IVF.
Table 13.2
Result of transplantation of embryo to recipient mouse cultured on the gate of the semiconductor and the culture
Number of transferred embryo | Number of offspring | Rate of offspring (%) | |
---|---|---|---|
Culture on gate of semiconductor | 20 | 10 (♂6, ♀4) | 50 |
Culture on dish | 20 | 8 (♂5, ♀3) | 40 |
The detection principle of the eSET-FET sensor is based on the potentiometric detection of charge density changes at the gate insulator, on which specific binding between target and probe molecules is made for molecular recognition. Figure 13.13 shows the conceptual structure of the eSET-FET sensor for detection of embryo activity after IVF. A single mouse embryo was set on the gate sensing surface. The gate surface of the eSET-FET sensor is immersed in a measurement solution together with a platinum (Pt) reference electrode (Fig. 13.12c). Basically, ionic or molecular charges at the gate interact electrostatically with electrons in the silicon crystal through the thin gate insulator and induce electrical signals by the field effect, resulting in the source and drain current (I D) change at the channel (Fig. 13.13a). Eventually, the output voltage is monitored as the shift of surface potential using a circuit (Fig. 13.13c) with which the potential change at the interface between an aqueous solution and the gate insulator can be read out directly at a constant I D. The charge density changes based on hydrogen ions can be detected as the shift of surface potential of eSET-FET sensor, because a hydroxyl group reacts equivalently with a hydrogen ion with positive charge according to pH (Fig. 13.13b). Here, this measurement principle is found to be noninvasive for an embryo culture. The details of the eSET-FET sensor and the fabrication process have been reported previously by our group. Furthermore, two types of eSET-FET sensors were prepared for differential measurements in this study: one was the eSET-FET sensor on which a single mouse embryo was kept and the other was the control FET sensor without an embryo (Fig. 13.12a). Using them, differential measurements were performed in order to eliminate the common unexpected signals such as temperature change, nonspecific adsorption, and change in ion concentration.
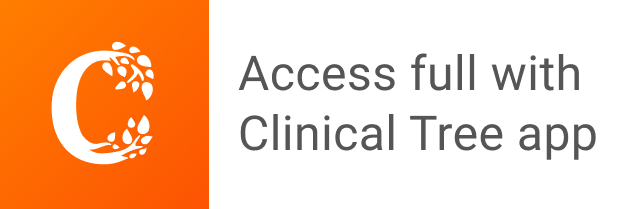