Fig. 11.1
CLSM images of CG (a), C-PG (b), CH-G (c), and CH-PG (d) particles (Modified from Wei et al. [107] with permission from John Wiley and Sons
Besides emulsification, the method of precipitation also has been used to prepare hydrogel particles, especially for those particles in nanoscale [8, 25, 44]. To induce the precipitation of polymer, there are mainly two methods, including adding non-solvent or the agent with opposite charges. Masotti et al. used both of the methods to prepare chitosan nanoparticles [53]. The methanol was used as non-solvent to precipitate chitosan, and the average diameter of formed nanoparticles was 45 ± 9 nm. DNA was added as anionic agent and formed nanoparticles with chitosan with an average size of 38 ± 4 nm. For preparing chitosan nanoparticles, tripolyphosphate (TPP) is the ionic cross-linking agent with the highest frequency of utilization [15, 56, 67]. By varying the composition and preparation conditions, the nanoparticles with different characteristics, such as size and surface charge, can be obtained [24].
Besides chitosan, alginate has also been frequently used to prepare hydrogel particles by precipitation. Anionic alginate tends to form “egg-box junctions” with multivalent cations, especially Ca2+ [86]. The formed Ca-alginate particles usually have an average diameter of nanoscale and been used for gene delivery and bone repair [88, 117]. But for some special applications, precipitation has also been used to prepare particles with bigger size. For example, in order to treat phenylketonuria (PKU) patients, which is an inherited metabolic disorder, Lactococcus lactis-expressing phenylalanine ammonia-lyase (LLEP) is proved effective. However, LLEP tends to lose its bioactivity in the stomach. Thus, in order to develop its oral formulation with proper bioactivity, entrapped LLEP in particles seems to be a good choice. The average size of LLEP is about 1 μm, and to entrap LLEP in particles, the particles should be in micrometer range. Zhang et al. used precipitation method to prepare LLEP entrapped alginate microparticles by combining with spray-solidification technique, which is to spray alginate solution that contained LLEP directly into CaCl2 solution [121]. The formed Ca-alginate microparticles have an average diameter around 58–59 μm as shown in Fig. 11.2.


Fig. 11.2
Optical images of LLEP entrapped alginate microparticles (Reproduced from Zhang et al. [121] with permission from ACS Publications)
However, as Fig. 11.2 shows, the shape of these microparticles is irregular and their size distribution is broad. For particles prepared for biomedical application, their size and shape are very important factors [87]. Particles with different diameters tend to aim to different organs when they are used as drug delivery system, which decreases the bioavailability of the entrapped drug [21]. Moreover, the size of particles affects their transmembrane efficiency when the particles are used as oral drug delivery system. Wei et al. fed the Sprague–Dawley (SD) rats with chitosan particles with different sizes (2.1, 7.2, and 12.5 μm) [108]. The bioadhesive ability of chitosan particles in the gastrointestinal tract was determined by modified everted sac experiment combined with flow cytometry analysis. By counting the number of particles in per mg tissue, it was found that more particles with 2.1 μm adhered on the mucus due to their small size (Fig. 11.3). Furthermore, the particles with small size (2.1 μm) could penetrate the mucosal membrane and be absorbed in the gastrointestinal tract, while those bigger particles (12.5 μm) could not. This study proved the importance of size control of particles as drug delivery system.


Fig. 11.3
Bioadhesion of chitosan microparticles with different diameters in the stomach (a) and the intestinal tract (b) (means ± SD, n = 6). AGJ means artificial gastric juice (hydrochloric acid solution, pH 1.2); PBS means phosphate-buffered saline (pH 7.2) (Reproduced from Wei et al. [108] with permission from Elsevier)
Thus, in order to obtain particles with narrow size distribution, several novel preparing methods were developed, and Shirasu porous glass (SPG) membrane emulsification technique is one representative of them. The SPG membrane is consisted of Al2O3–SiO2 and has uniform-sized pores on its wall, so by pressing the dispersed phase through the SPG membrane into continuous phase, the emulsion with uniform-sized droplets can be obtained. The principle of SPG membrane emulsification is illustrated in Fig. 11.4 [99].


Fig. 11.4
Principle of SPG membrane emulsification
Wang et al. used SPG membrane emulsification to prepare chitosan microparticles, and the number-average diameter of prepared particles is 13.8 μm with coefficient of variation (CV) value below 15 % [99]. The scanning electron microscopy (SEM) photograph of chitosan microparticles is shown in Fig. 11.5.


Fig. 11.5
SEM photographs of chitosan microspheres prepared by membrane emulsification technique (Modified from Wang et al. [99] with permission from Elsevier)
Besides chitosan particles, agarose [125, 126], alginate [122], and poly(N-isopropylacrylamide-co-acrylic acid) [P(NIPAM-co-AA)] hydrogel particles [101] prepared by SPG membrane emulsification technique were reported, and all of these formed particles have narrow size distribution.
However, by this SPG membrane emulsification technique, which is also called general SPG membrane emulsification technique, the diameter of formed droplets is several times bigger than the pore size of used membrane. So in order to prepare particles in nanoscale, the membrane with very tiny pores should be adopted, which would dramatically lower the productivity. And for some viscous systems, the preparation process would be very difficult if not impossible.
To remedy these disadvantages, a novel SPG membrane emulsification, premix SPG membrane emulsification, was developed. In this technique, the dispersed phase is firstly mixed with the continuous phase by stirring, homogenizing, or other methods, and the mixed solution is called coarse emulsion or pre-emulsion. Then, the coarse emulsion is pressed through SPG membrane one or several times by nitrogen gas to obtain droplets with narrow size distribution. The principle of premix SPG membrane emulsification is shown in Fig. 11.6.


Fig. 11.6
Principle of premix SPG membrane emulsification
By premix SPG membrane emulsification technique, it is probable to prepare uniform-sized particles with nanoscale or for systems with high viscosity. For example, agarose, the natural polysaccharide, has high viscosity and been widely used as chromatography medium. In order to separate biomolecules more quickly and efficiently, small agarose beads are desirable due to their big surface area. However, the reduction of bead size results in small spaces among the beads and high column back pressure. In order to resist the high pressure, one way is increasing the agarose content of beads. But, with the increase of agarose content, the water phase becomes very viscous, so that it is very difficult to prepare uniform-sized agarose beads with high agarose content and small size by stirring or other common techniques. The development of premix SPG membrane emulsification solved this problem. By this technique, agarose beads with high polymer content (14 wt.%) and small diameter (less than 10 μm) have been prepared with CV of 9.8 % [127, 128]. And for other systems with high viscosity, this technique still works. Lv et al. used this technique to prepare chitosan and N-((2-hydroxy-3-trimethylammonium) propyl) chitosan chloride (HTCC) nanoparticles with polydispersity index 0.029 and 0.034 separately [51].
Microchannel (MC) emulsification technique is another useful method to produce monodisperse droplets or particles [38, 69]. The main preparation process is pumping the dispersed phase into continuous phase through special silicon plate, stainless steel, or PMMA array with predesigned microgrooves or microchannels (Fig. 11.7), and the typical structure of silicon array is illustrated in Fig. 11.8.



Fig. 11.7
Microchannel emulsification experimental setup based on silicon plate (Modified from Chuah et al. [13] with permission from Elsevier)

Fig. 11.8
Silicon MC array with microgrooves (left) or with straight – through microchannels – (right)
The size of produced droplets is mainly affected by the size of the microgrooves or microchannels, the flow rate of dispersed phase, and the compositions of both dispersed phase and continuous phase. By optimizing these factors, uniform-sized particles can be obtained. For instance, Chuah et al. prepared monodisperse alginate microspheres with an average size of 6.2 μm and CV of below 10 % by MC emulsification [13]. Besides alginate microspheres, gelatin microbeads [32], poly(N-isopropylacrylamide) (PNIPAM) microparticles [31], and chitosan microparticles [114] with uniform size have been fabricated successfully by MC technique.
Microfluidic (MF) technique is another important method that utilized microfabricated devices to control the size of formed droplets [16], which has been reported frequently in recent years. MF technique is divided into planar MF and three-dimensional axisymmetric MF. The main component of planar MF device is poly(methyl methacrylate) (PMMA) plate with microchannels on silicon mold. The structure of microchannels includes several types, such as T junction, Y junction, and cross junction (Fig. 11.9).


Fig. 11.9
Typical structures of microchannels of planar MF devices: (a) T junction, (b) Y junction, and (c) cross junction
These special structures of microchannels provide the possibility to prepare particles with various structures or morphologies. For example, Hwang et al. used MF device with T junction structure and prepared nonspherical hydrogel microparticles with ɤ-Fe2O3 nanoparticles inside [30]. The dispersed phase (magnetic solution) and continuous phase (mineral oil) were independently pressed by air through the microchannels to form emulsion. And by adjusting the channel geometries, pressure for dispersed phase and continuous phase, microgels with different morphologies were prepared, including spheres, disks and plugs.
Another type of anisotropic microparticles, Janus particles, can also be prepared by MF technique. Janus particles have hemispherically distinct geometries as shown in Fig. 11.10 [97]. Due to their unique anisotropy, the preparation and application of Janus particles have been reported in many literatures [11, 59, 63].


Fig. 11.10
Different types of Janus particles (JPs): spherical (a), two types of cylindrical (b, c), and disk-shaped (d, e) JPs. (f–k) Various kinds of dumbbell-shaped JPs with asymmetric or snowman character (f), symmetric appearance (g, k), attached nodes (h), and eccentric encapsulation (i). (l) Janus vesicles or capsules (Reproduced from Walther and Müller [97] with permission from ACS Publications)
For Janus particles, whether they have narrow size distribution is important for their biomedical application. By using MF device with Y junction structure, Aketagawa et al. prepared monodisperse Janus hydrogel beads with CV of less than 5 % [1]. Two different dispersed phases (sodium alginate solution containing sodium citrate and sodium alginate solution containing Fe3O4 nanoparticles) were separately injected through the two inlets of Y junction slowly to form a two-phase parallel stream (Fig. 11.11). After entering into a continuous phase, biphasic droplets were formed. At the presence of electrolyte, the Fe3O4 nanoparticles aggregated and precipitated at the bottom of the droplets. Then, the Janus alginate microparticles with magnetic anisotropy were prepared.


Fig. 11.11
Fabrication of alginate microparticles with magnetic anisotropy by MF device with Y junction structure (Reproduced from Aketagawa et al. [1]. (Open Access))
However, for these planar MF devices, the formed droplets have to contact the wall of the microchannels during formation and flow, this may damage the formed structure of some fragile particles [96]. In order to conquer this problem, a novel three-dimensional axisymmetric MF device was developed. A classic example of axisymmetric MF device is glass capillary device as shown in Fig. 11.12.


Fig. 11.12
Axisymmetric glass capillary devices: (a) co-flow of two immiscible fluids, (b) countercurrent flow of two immiscible fluids with flow focusing, (c) combination of co-flow and countercurrent flow of three immiscible fluids, (d) two sequential co-flow droplet generators, (e) injection of two distinct inner phases of double emulsions using a two-bore injection tube (Reproduced from Vladisavljević et al. [96] with permission from Springer)
In glass capillary device, the dispersed phase is surrounded by the continuous phase, which avoids the contact between newly formed droplets and the wall of microchannels. Moreover, by designing glass capillary devices with different structures, the droplets and microparticles with various structures or morphologies can be prepared easily as shown in Fig. 11.13 [12, 14, 48, 77, 102, 104].


Fig. 11.13
Particles with various structures prepared by MF technique (Reproduced from Wang et al. [102] with permission from ACS Publications)
As three promising techniques, SPG membrane emulsification, MC, and MF technique, all of them show their advantages to provide droplets or particles with narrow size distribution over other common methods. Moreover, these techniques are energy efficient because they do not need high energy to disperse the emulsion. Comparing these three methods, MC and MF techniques are superior to SPG membrane emulsification on the size distribution, structure, and shape control of particles, while the latter shows its advantages on the large-scale production [96]. Furthermore, SPG membrane emulsification technique can be utilized to prepare submicro- and nanoparticles. But for MC and MF techniques, due to the limit of the processing technology, the size of microchannels is at least several micrometers; thus it is hard to utilize MC and MF techniques to prepare nanoparticles. However, in spite of these techniques having been proved useful for uniform-sized particle preparation, they have one common shortcoming. All of these techniques utilized a membrane, plate, or tube with microchannels as their key component; for preparing the dispersed phase with high viscosity or solid granules, the microchannels tend to be blocked which hinders the preparation process or even leads to failure.
In recent years, there are still other techniques which have been introduced to prepare uniform-sized hydrogel particles, such as ink-jet printing [19, 33], imprint lithography [9], and microfluidic electrospray technique [49]. Some of them show their advantages in size distribution and structure control of particles. With more intensive study, these techniques may play a greater role in the preparation of hydrogel particles.
11.3 Characterization
Like other polymer particles, the size and size distribution, surface characteristics, morphology, and interior structure of hydrogel particles are important factors for their applications. For accurate measurement, it needs to be taken in consideration that hydrogel particles have some special characteristics which would affect their characterization.
For example, most of the hydrogel particles have high water content, and some particles even contain more than 90 % (w/w) of water. Due to their high water content, the hydrogel particles have porous structure, which facilitate the adsorption and diffusion of biomacromolecules. But it is difficult to characterize the porous structure of hydrogel particles because of their weak mechanical strength. The commonly used method for pore structure characterization, mercury intrusion porosimetry, needs high pressure, which might crush or deform the particles [5]. Before using the scanning electron microscopy (SEM) to observe the morphology of particles, the samples have to be dried, and the drying process might damage the gel network as stated before [54]. In order to keep the original structure of hydrogel particles, Du et al. adopted critical point drying to dry agarose beads [20]. Although the drying process is very mild, the particles still shrink and the porous structure of the hydrogel could not be observed clearly due to dehydration.
To avoid the effect of the drying process, the techniques which can test the samples in liquid environment have been investigated for characterization of hydrogel particles. Atomic force microscopy (AFM) and confocal laser scanning microscopy (CLSM) are two representative techniques among them. The measurement conducted by AFM can achieve high spatial resolution of the surface morphology of samples [4, 41]. Seeber et al. utilized AFM to observe surface morphology change of the thermosensitive microgel below and above its lower critical solution temperature (LCST) [76]. The swell and collapse of microgel can be observed and measured by detecting the change of layer thickness.
However, in order to measure accurately, the particles need to be fixed onto a substrate. Usually electrostatic attraction between particles with substrate is utilized to fix the particles. But for some samples uncharged or with weak charges, chemical cross-linking has to be used, and the cross-linking may affect the structure of hydrogel particles. Moreover, for soft hydrogel particles, the force of AFM probe may cause the deformation of particles and the obtained results are not so reliable, especially for the measurement of height [9].
CLSM is another technique for obtaining optical images of hydrogel particles in liquid surroundings. The key feature of CLSM is its ability to observe the interior structure without the need of destroying the samples. Yang et al. prepared superporous agarose (SA) microspheres and used CLSM to prove the existence of pores (Fig. 11.14) [115]. It is clearly observed from Fig. 11.12c, d that inside of SA microspheres, there are some fluorescence signals, which proves fluorescein isothiocyanate (FITC)-labeled IgG (FITC-IgG) can enter the interior of microspheres through the superpores. While for those homogeneous agarose (HA) microspheres, without the existence of superporous structure, FITC-IgG cannot enter the interior of microspheres and only binds with protein A on the surface of microspheres (Fig. 11.12a, b).


Fig. 11.14
Surface availability on HA and SA microspheres for binding with protein A. (a, c) Sectional images were obtained from the middle of HA (a) and SA (c) beads by CLSM. (b, d) Graphical representations of the fluorescence intensities were obtained from (a) and (c) images, respectively (Reproduced from Yang et al. [115] with permission from Elsevier)
CLSM can also be utilized to observe the diffusion of molecules in hydrogel particles. Wu et al. prepared chitosan hydrogel microparticles and entrapped FITC-labeled bovine serum albumin (BSA) as a model drug inside [111]. With the release of drug, the fluorescence intensity of microparticles decreased. However, in order to characterize by CLSM, the tested samples need to be fluorescence labeled, and the pretreating process is relatively complex and time-consuming.
Besides the porous structure, the mechanical strength is another important property of hydrogel particles. Conventional hydrogels have weak mechanical strength and they tend to deform or be broken under compression. But for some applications, whether the particles have essential high mechanical strength is important. For instance, the particles used as separation medium must have mechanical strength high enough to keep their integrity under operating pressure. Otherwise, these particles will collapse, and the deformed particles tend to block the gaps between particles, which lift the column back pressure rapidly and may lead to the failure of separation. In order to measure their mechanical strength, one method is measuring the pressure–flow rate curve of particles in the column. By this method, the tested particles need to be packed in the column and the flow rate of solution (usually water) though this column is measured constantly. With the increase of pressure up to a point, the particles deform or collapse and the column back pressure increases sharply. However, for this method, amount of samples needs to be used. In order to reduce the amount of samples, several techniques, which can detect the mechanical strength of individual particle, have been proposed, including AFM [6, 57, 116] and microelectromechanical systems (MEMS) [37]. Micromanipulation technique is another one which detects the mechanical strength of a particle in microscale. Yan et al. used this technique to test individual agarose microsphere [113]. The equipment is shown in Fig. 11.15. Single microsphere is compressed by a probe controlled by a force transducer. Then, by measuring the change of force and the diameter of the microsphere, the mechanical strength of the microsphere is calculated.


Fig. 11.15
Schematic diagram of the micromanipulation rig: (1) force transducer, (2) probe, (3) stepping motor, (4) computer with a data acquisition board, (5) lower-view microscope, (6) side-view microscope, (7) video recorder, (8) agarose microsphere in water, and (9) glass chamber (Reproduced from Yan et al. [113] with permission from Elsevier)
11.4 Application
11.4.1 As Drug Delivery System
Most of the literatures related to the application of hydrogel nano- and microparticles in biomedical field focus on drug delivery system. As other delivery systems are based on particles, the utilization of hydrogel particles helps to protect loaded drug from degradation of enzyme, acid, or other adverse surroundings in vivo. In addition, hydrogel particles have some special characteristics, which facilitate their biomedical application. Firstly, hydrogels contain high percent of water, and the presence of water improves the biocompatibility of hydrogels and makes them nonirritant for entrapped biomacromolecules. Thus, the introduction of hydrogels will help keep the bioactivity of entrapped drugs. For example, Wang and Wu combined agarose hydrogel particles with poly(lactic-co-glycolic acid) (PLGA) microspheres to stabilize entrapped protein drugs [98]. The model drug, insulin, was encapsulated in hydrogel particles and then dispersed in PLGA microspheres. For sole PLGA microspheres, the encapsulation process, the hydrophobicity of PLGA, and the acidic microenvironment due to the polymer degradation induced the degradation and aggregation of insulin, and about 18 % insulin in PLGA microspheres was denatured. But after entrapping drugs in the agarose particles and dispersing these particles in PLGA microspheres, only 1 % denatured insulin was detected in the release medium. The high retention efficiency of drug bioactivity was attributed to drug encapsulation by agarose particles, which isolated drug from the aqueous–organic interfaces and hydrophobic and acidic microenvironment. Schoubben et al. carried out another similar study [74]. They introduced alginate particles into PLGA microparticles and found the addition of alginate particles stabilized the entrapped insulin and prolonged the release period up to 4 months.
Secondly, although the main function of drug carriers based on particles is releasing drug slowly and constantly to prolong the half-life of drug in blood circulation, some of hydrogel particles have more functions than that. These hydrogel particles possess stimuli responsibility, which means that they change their shape, size, or other characteristics responding to external stimuli, such as temperature, pH, and so forth. Depending on this special property, these hydrogel particles have been developed as injectable drug delivery system, targeting drug delivery system, and stimuli-responsive delivery system [58, 71, 92, 122].
In order to obtain injectable systems, the thermosensitivity of hydrogels would work. For example, Hsiao et al. designed thermosensitive chitosan nanocapsules and developed injectable depot delivery system based on these nanocapsules [28]. They synthesized carboxymethyl-hexanoyl chitosan (CHC), firstly, and CHC would self-assemble to nanocapsules. With the adding of negatively charged sodium β-glycerophosphate (β-GP), these nanocapsules showed thermosensitivity, that is, they transformed from solution state into gel at body temperature. Antiepileptic drug ethosuximide was loaded as model drug in this system and the in vivo study proved its treating efficiency.
For developing targeting drug delivery systems, the hydrogel nano- and microparticles with pH sensitivity have been frequently investigated. Chitosan, the positively charged polysaccharide, and alginate, the negatively charged polysaccharide, are the most commonly used materials to prepare pH-sensitive particles for colon-targeting drug delivery or tumor-targeting drug delivery. Li et al. fabricated nanoparticles composed of quaternized chitosan and alginate [43]. These nanoparticles showed pH-dependent drug release profiles. BSA as a model drug was released slowly in simulated gastric fluid (pH 1.2) and rapidly in simulated intestinal fluid (pH 7.4).
The positive charge of chitosan not only helps the colon-targeting drug delivery but also promotes the drug absorption through the mucosal membrane. Wei et al. fabricated an oral delivery system for siRNA based on N-((2-hydroxy-3-trimethylammonium)propyl) chitosan chloride (HTCC) nanoparticles (HNP) [110]. The positive charge of HNP facilitated the permeation of siRNA in the intestine tract as shown in Fig. 11.16. By co-encapsulating siRNA and paclitaxel (PTX) in HNP, the complex system could achieve effective tumor suppression effect.


Fig. 11.16
Absorption of siRNA (left) and HNP:siRNA (right) in the small intestine. Scale bar: 100 μm (Modified from Wei et al. [110] with permission from Elsevier)
Moreover, the stimuli responsibility of hydrogel particles can be utilized to realize special release profiles, like trigger release. Inspired by the natural plant, squirting cucumber, Liu et al. prepared thermosensitive hydrogel capsules and designed an interesting system based on these capsules for nanoparticle delivery with thermo-triggered squirting property [47]. The shell of hydrogel capsules is composed of PNIPAM, which would shrink dramatically at the temperature above its LCST. By the aim of microwave or infrared irradiation, when the capsules were heated above the LCST of PNIPAM, their shell would shrink rapidly and result in squirting delivery of entrapped nanoparticles.
Besides the stimuli of pH and temperature, biomolecules have also been used as stimulus to regulate the drug release rate from hydrogel particles. For example, to treat diabetes I, the patients need to inject insulin two or three times every day. In order to avoid the daily injection and keep the blood glucose level in normal range, glucose-responsive microcapsules have been promoted. Zhang et al. fabricated monodispersed glucose-responsive hydrogel microcapsules by UV-initiated polymerization of glucose-responsive 3-acrylamidophenylboronic acid (AAPBA) and thermo-responsive PNIPAM as the microcapsule shell [123]. These microcapsules exhibit reversible swelling/shrinking behavior and different drug release rate responding to glucose concentration changes.
Multi-stimuli-responsive hydrogel particles have also been reported. Sahiner et al. designed hydrogel nanoparticles with core–shell structure and magnetic Fe3O4 inside of them [70]. These particles are sensitive to pH change and magnetic field. Moreover, these particles could be further modified with other functional groups or combined with other metals to achieve more stimuli responsibility.
Thirdly, hydrogel particles usually have porous network structure and high permeability for drug, ions, or other water-soluble metabolites, which make them attractive candidates for use in the biomedical field. With the porous structure, hydrogel particles can load more drugs than solid polymer particles. Moreover, by changing the porous structures, the different drug release profiles would be obtained to fulfill various treatment requirements. Wei et al. designed four types of chitosan microspheres with different structures and investigated their drug release profiles (Fig. 11.17) [107]. The solid particles (CG) released drug with high initial burst because most of the drug is dispersed near the particle surface. And C-PG microspheres with hollow structure showed triphase drug release profile. The diffusion of drug in the particle shell resulted in the first burst release, and then the drug in the particle cavity is diffused outside. After that the concentration gradient of drug in the microspheres dominated the last slow release. The particles with hollow structure and pores on the surface (CH-G) released drug with minimal initial burst and would be beneficial for controlled release of protein drugs. The particles with macroporous structure (CH-PG) showed a strong initial burst, and the authors suggested using these particles for pulsed therapy, for instance, vaccine administration.


Fig. 11.17
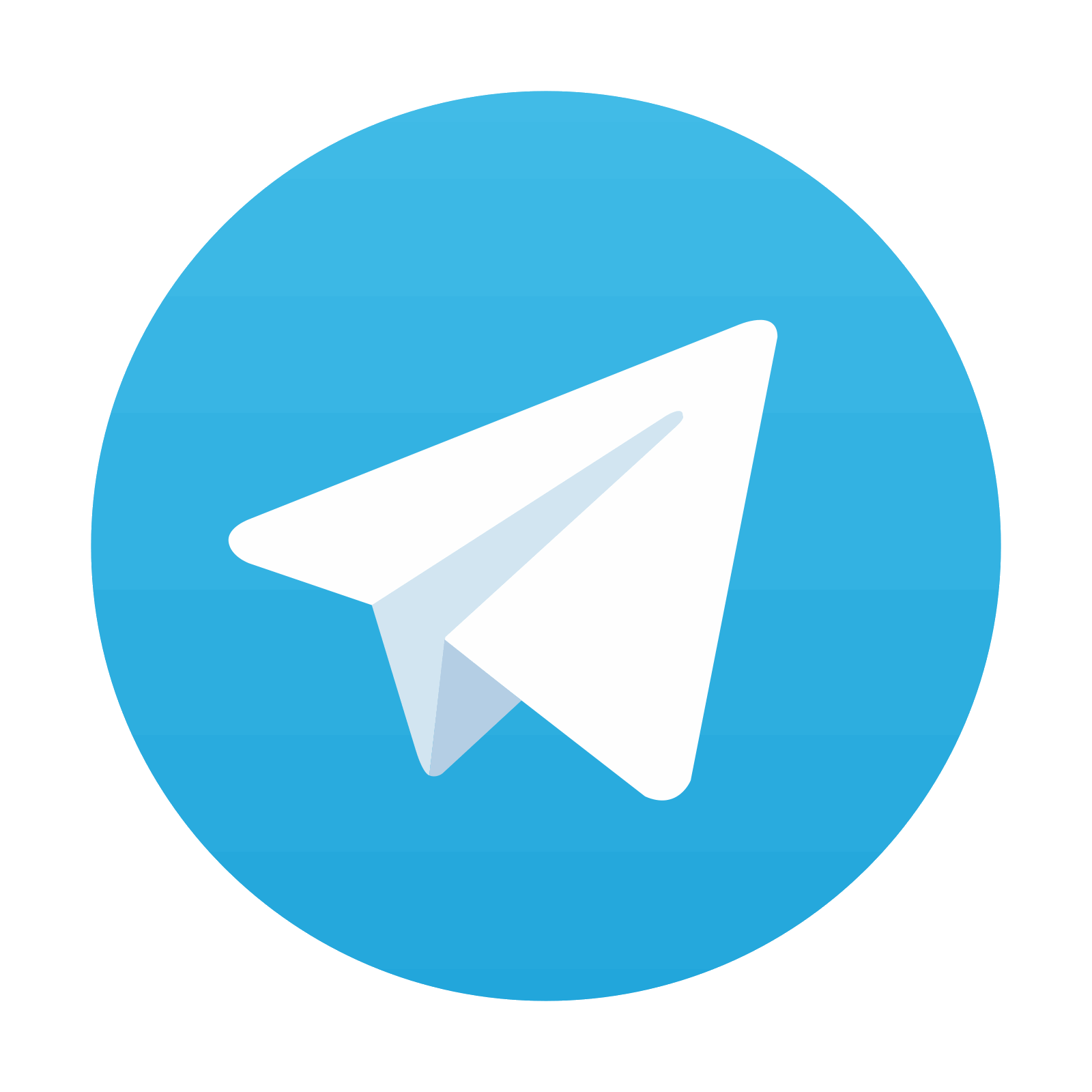
Schematic representation of BSA (green dots) distribution in microspheres prepared by different methods: (a) CG microspheres, (b) C-PG microspheres, (c) CH-G microspheres, (d) CH-PG microspheres, and (e) their different release profiles (Modified from Wei et al. [107] with permission from John Wiley and Sons)
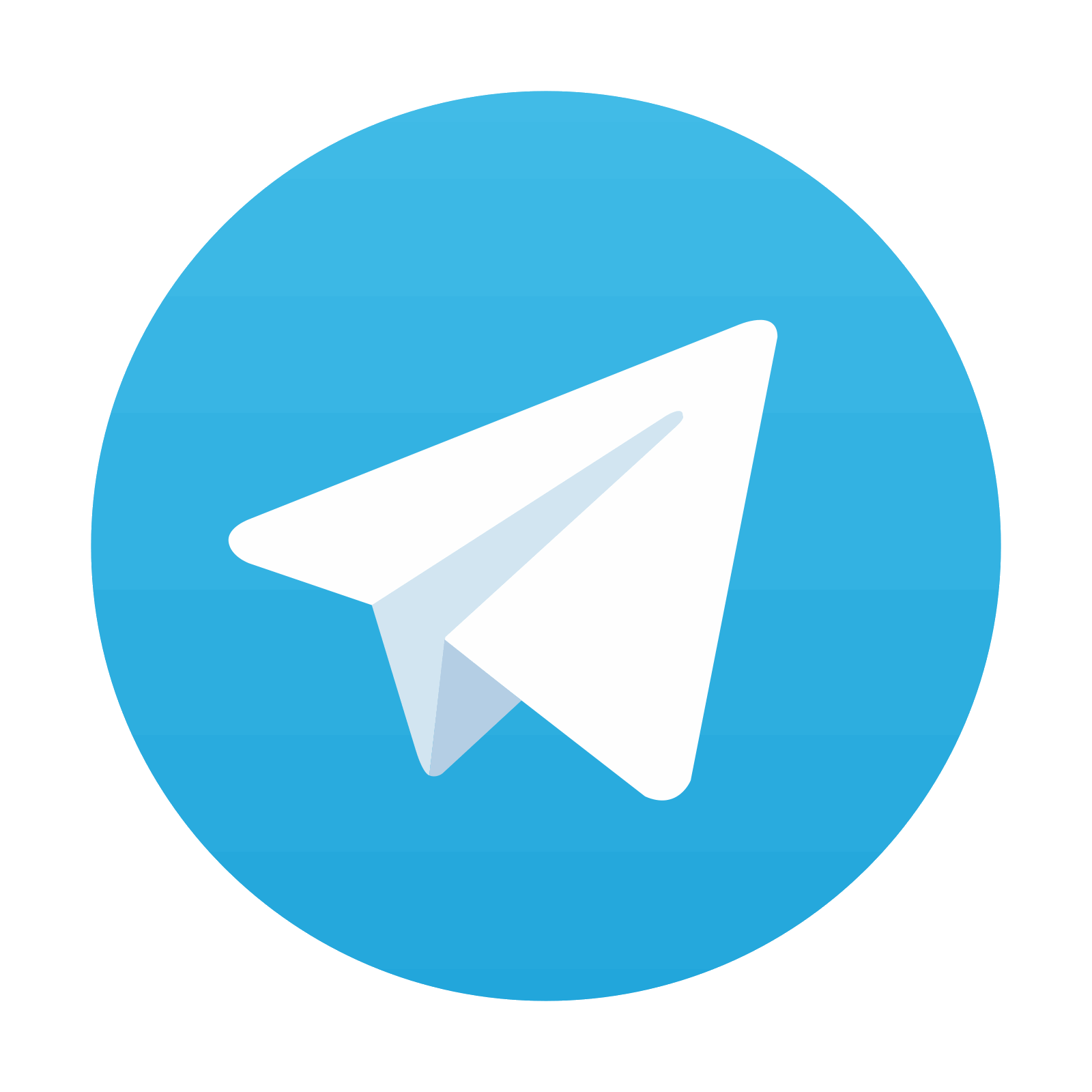
Stay updated, free articles. Join our Telegram channel
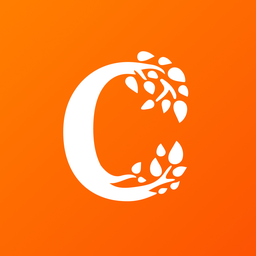
Full access? Get Clinical Tree
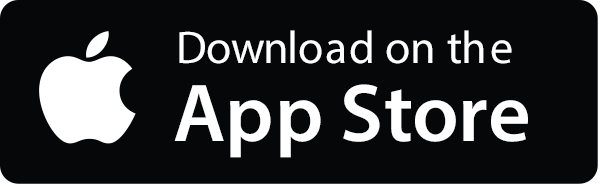
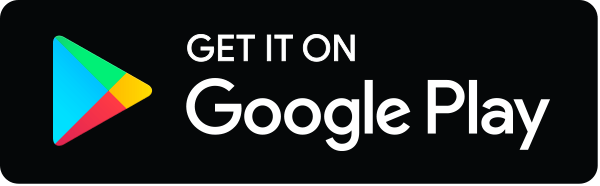
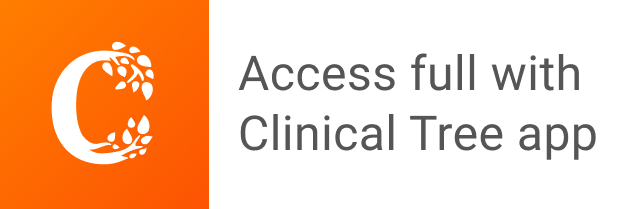