A. IMPORTANCE OF SEMICONDUCTORS
Semiconductors are found in virtually every technological device, from computers, telephones, radios, light-emitting diodes, lasers, and television screens to medical sensors, smoke detectors, cameras, and automobile and aircraft controls. Crude semiconductor devices were first introduced in the 1950s, but the subsequent incorporation of hundreds to millions of semiconductor units onto a small area the size of a fingernail has been one of the major triumphs of modern science and technology. Probably no single material has had a greater influence on our way of life in the last hundred years than the semiconductor.
Semiconductors are solids that conduct electricity at a level that falls between those of an insulator and a metal—typically between about 10−8 S/cm (siemens per centimeter) to around 1 S/cm. Many different solid materials fall into this category. Examples include not only well-known substances such as silicon or germanium, and compound semiconductors such as gallium arsenide or cadmium sulfide, but also certain unsaturated organic polymers such as polyacetylene and poly(phenyleevinylenes). Although the inorganic semiconductors (such as silicon) have traditionally dominated the electronics field in the past, polymers are beginning to be used for special applications where materials flexibility and relatively low cost of production are important.
Most semiconductor materials need to be modified by the introduction of a “dopant” before their electronic behavior can be tailored for useful applications. A dopant is typically a small-molecule compound or element that donates electrons to the host material (an n-type semiconductor) or removes electrons from the host (a p-type semiconductor). Typical dopants are minute quantities of elements such as boron or phosphorus added to silicon, or larger quantities of compounds such as iodine or arsenic pentafluoride that are added to organic polymeric semiconductors. The role of dopants is discussed in the following pages. In subsequent sections we consider semiconductor theory, the preparation of semiconductor-grade materials, the fabrication of integrated circuits, and some applications of semiconductors.
The most important defining feature of a semiconductor is its bandgap. As discussed earlier, the electronic structure of a conductive material is best described in terms of energy bands rather than individual orbitals. The result of combining millions of orbitals into a band structure is the formation of a lower-energy valence band derived from all the bonding orbitals of the participating atoms, and a conduction band derived from all the antibonding orbitals. These are separated by a bandgap, which varies from substance to substance (Figure 10.1). As discussed in Chapter 8, metals have a partly filled valence band and an empty conduction band. Electronic conduction occurs by excitation of electrons to levels in the unfilled region of the valence band. Insulators have a filled valence band, an empty conduction band, and a high-energy gap between them. On the other hand, semiconductors have a filled valence band and an empty conduction band, but the two bands are separated by a relatively small energy gap in the range of 1 eV. The bandgaps of some different solids are listed in Table 10.1.
Electrical conduction in an inorganic semiconductor occurs through the movement of either unpaired electrons and/or holes. A “hole” is the positively charged entity that is left when an electron is removed from an electron pair. Unpaired electrons can be formed in two ways. First, exposure of the material to heat or light can cause electrons in the upper levels of the filled valence band to jump the bandgap into the lowest levels of the conduction band. Once promoted in this way, they are free to move through the solid under the pressure of an external negative electrical potential. The positive holes that are left behind in the valence band are also free to move, but in the opposite direction from the electrons. Thus, current passes via these counterflowing streams of charged units. However, the flow of holes and electrons may not be equal. Some semiconductors conduct primarily through the movement of electrons and others, mainly through hole migration. The situation that prevails depends on the solid structure and the elements involved. For example, the ratio for the relative mobility of electrons versus holes in silicon is 3:1. In indium phosphide it is 33:1.
TABLE 10.1. Bandgaps of Different Solids (e V)

The second mechanism that underlies the behavior of semiconductors involves the dopants or impurities that are distributed throughout the lattice. Silicon may be doped with either an element that lies in group III (13) of the periodic table, such as boron, or with an element such as phosphorus or arsenic in group V (15). The addition of each group V element to the silicon lattice adds an extra electron because the group V elements have one more electron in the valence shell than does silicon. Where does this electron go? It goes into a new energy level in the bandgap (Figure 10.2b), from which it can be promoted easily by the absorption of heat or light energy into the conduction band. Conversely, the presence of a group III element impurity creates a new energy level in the gap into which an electron can jump from the valence band (Figure 10.2a). Thus, the impurities serve to lower the actual bandgap and provide additional electrons or holes.
What would happen if equal amounts of both boron and phosphorus were added as impurities to the lattice? Very little, because the two would cancel each other out with respect to adding electrons or holes to the system. However, both n and p dopants may be used in adjacent areas of the same semiconductor for ease of integrated circuit fabrication. It is sometimes easier to start with, say, an n- or p-doped material and then add a smaller or larger amount of the opposite dopant to gain a close control over the amount of impurity introduced and the exact level of conduction.
This raises another question. How does this apply to compound semiconductors, such as gallium arsenide or gallium phosphide, in which the whole solid lattice is composed of equal amounts of group III and group V elements held together by a combination of ionic and covalent bonds? Unlike silicon, these materials are direct-bandgap semiconductors, which are the favored materials for light emission from light-emitting diodes or semiconductor lasers. Do these materials need to be doped, or do they already have their own built-in dopant in the lattice? The answer is that a “dopant” is still needed. It may take two forms: (1) GaAs, for example, can be p-doped by the addition of a group II element such as berylium or zinc, or n-doped by a group IV element such as carbon or silicon, or an excess of gallium or arsenic will serve the same purpose; or (2) the doping can be in the form of a third element that gives rise to variable-composition alloys. This third element controls the bandgap. This is important because these materials are the mainstay of the light-emitting diode and laser diode industries, and the added element controls the color of the light emitted when an electric current is passed through the solid. These materials are described by a formula such as GaAs1-xEx, where E is the dopant element. Thus, GaAs1-xPx is widely used in LEDs, with the amount of phosphorus controlling the color of the light emitted. For example, GaAs itself emits in the infrared but, as an increasing amount of phosphorus replaces the arsenic, the emitted color changes to red and eventually to green. Other colors, such as blue or violet are generated when nitrogen or tellurium are introduced.
Figure 10.2. (a) Incorporation of B or Ga into silicon generates a new energy level in the lower region of the bandgap into which mobile electron from the top of the valence band can readily jump. (b) Doping of silicon with P or As inserts electrons into a new energy level in the upper region of the bandgap, from which electrons can easily jump to the lower levels of the conduction band. Both processes lower the effective bandgap and lead to increased conduction.

C. PREPARATION OF SEMICONDUCTOR-GRADE SILICON AND COMPOUND SEMICONDUCTORS
1. Semiconductor-Grade Silicon
As mentioned in Chapter 3, the manufacture of pure silicon is an involved process that requires first a reduction of sand by carbon to give a mixture of silicon and silicon carbide, followed by reaction with chlorine to give SiCl4 and CCl4, which are then separated by fractional distillation. Treatment of the SiCl4 with a reactive metal such as zinc or magnesium or with hydrogen gives partially pure silicon. However, this is not nearly pure enough for semiconductor use. A final purification is effected by the process of pulling an ingot from a bath of molten silicon and/or zone refining (Figure 10.3).
Figure 10.3. Purification of silicon for semiconductor applications. (a) A cylindrical ingot of crystalline silicon is drawn from a molten silicon bath. (b) Zone refining by movement of a molten zone in which impurities are concentrated and deposited at one end of an ingot.

Ingot growth and zone refining are both based on the principle that the solid that crystallizes from an impure molten material is purer that the molten material. This occurs because the mechanism of crystallization and the growth of a crystal lattice tend to exclude any atoms or compounds that do not fit efficiently into the solid lattice. Thus, if a crystal of pure silicon is suspended at the surface of molten silicon, it will stimulate the deposition of additional pure material on the original crystal. As the solid material is pulled very slowly from the surface of the melt, an ingot of very pure silicon will be obtained. Zone refining involves taking an ingot of semi-pure silicon and moving a circular heating element vertically from one end to the other. As the hot zone (~1420°C) of the heating element passes up the rod, the silicon melts and then recrystallizes, a process that concentrates the impurities in the moving molten zone. After a number of passes of the hot zone, the impurities will be concentrated at one end of the ingot, while the rest of the silicon will be highly pure.
Purified ingots are then cut into thin wafers by means of a diamond saw, are polished to reduce surface roughness to less than a ten-millionth of a meter, and are then ready for microlithography. A typical modern ingot will have a diameter of 300mm (~12 inches), which will give wafers that allow the fabrication of up to 2000 integrated circuits per wafer.
2. Amorphous Semiconductor Silicon
The process described above for the preparation of pure crystalline silicon is expensive—too expensive for the large-scale production of large-area devices such as solar cells. An alternative approach is the use of amorphous silicon produced by chemical vapor deposition (CVD) on a flat surface. When silane (SiH4) is decomposed in a radiofrequency plasma, it yields fragments that range from silicon atoms to SiH, SiH2, and SiH3. If these are allowed to impinge on a flat surface such as glass or a polymer, amorphous silicon contaminated by Si—H species is deposited as a coherent film. Amorphous silicon, as its name implies, consists of a random, three-dimensional network of Si—Si bonds interrupted by dangling Si—H units. The hydrogen serves as a dopant, and the films are semiconductive. Their efficiency as semiconductors is quite low. For example, when used in solar cells, amorphous silicon devices have an energy conversion efficiency of only 4% compared to about 10% for crystalline silicon.
3. Preparation of Compound Semiconductors
Some of the most important semiconductor materials are variable-composition alloys that contain combinations of three elements such as gallium and arsenic with phosphorus, or gallium and nitrogen, with aluminum or indium. Compound semiconductors such as GaAs1-xPx can be prepared by heating the ingredients together in a sealed, evacuated tube. However, chemical vapor deposition of a mixture of organometallic derivatives of the elements is a more reproducible approach using, for example, a single crystal of GaAs as a “template” to control the crystal structure of the depositing material. As these materials are used mainly for LEDs, quite small crystals (~1 mm3) are satisfactory. A major additional use for these species is in diode or “double heterostructure” lasers, again manufactured by chemical vapor deposition, as discussed later in this chapter.
D. ORGANIC POLYMER SEMICONDUCTORS
1. Background—Polyacetylene
Until the 1970s polymers were considered to be electrical insulators. An unstable inorganic macromolecule, poly(sulfur nitride) [(SN)x] was known to conduct electricity, but this was considered to be a bizarre aberration. Several events served to change that picture. First, early attempts to polymerize acetylene by free-radical techniques yielded black, insoluble, but electrically conductive materials (reaction 1). But these reactions can be explosive, and there was no way to fabricate films or fibers from these materials. Then in 1967, a method was found to polymerize acetylene to a new form of polyacetylene, (CHCH)n (reaction 2) a material that looks more like a metal than a conventional polymer. This polymer forms silvery or gold-colored films that were shown to conduct electricity at a level typical of a weak semiconductor (10−8S/cm). However, when treated with reagents such as iodine. arsenic pentafluoride, or antimony pentafluoride, or when oxidized with sodium, lithium, or radical anions, or reduced electrochemically, polyacetylene becomes a modest metal-level conductor (105S/cm) in the same range as mercury. These dopants add or remove electrons from the solid polymer, and facilitate conduction by a still controversial mechanism. Other methods to produce polyacetylene are shown in reactions 3 and 4.
Doped polyacetylene films are flexible, and have an almost unique morphology. They consist of polymer chains organized into microfibrils, clustered into larger fibrils that are intertwined into a fiber mat (Figure 10.4).
Figure 10.4. Representation of the morphology of polyacetylene films. The high-surface-area porous structure is valuable for uses as electrodes. It also facilitates the entry of dopants into the material.

Since the 1970s a number of other unsaturated polymers have been shown to yield electronic semiconductors when doped. These include poly(phenylene vinylene), polyphenylenes, polypyrrole, polythiophene, and polyaniline (reactions 5–9). They are discussed briefly in the following sections.
2. Poly(phenylene vinylene)
Although the electrical behavior of polyacetylene proved to be a groundbreaking discovery, uses for this polymer are severely limited by its instability to oxidation in the atmosphere and its insolubility. This restricts the options for device design and fabrication. Thus, the emphasis has shifted to studying other polyunsaturated polymers such as poly(phenylene vinylenes) or polythiophene, which are more stable in the atmosphere and are soluble in some organic solvents. Like polyacetylene, poly(phenylenevinylene) is an insoluble polymer and therefore cannot be fabricated by solution-casting techniques. Instead, a soluble polymeric precursor in first synthesized and fabricated, and a final reaction converts this to the required polymer. A typical process is illustrated by reaction 5.
Although poly(phenylene vinylene) itself is insoluble, the linkage of organic side chains to the main skeleton improves the solubility considerably, but at the expense of causing twisting of the chains and reduction of electron delocalization. Moreover, changes to the repeating unit structure bring about a change in the bandgap and the wavelength of the light emitted in electroluminescent devices. This is a crucial requirement for semiconductors used for display applications. A major use for poly(phenylene vinylenes) is in flat panel light-emitting devices, as described later in this chapter.
3. Poly(p-phenylene)
In principle, this polymer (reaction 6) when doped should be an excellent polymeric semiconductor due to the opportunities that exist for long-range electron delocalization and the stability of the polymer in air. In practice, certain constraints apply, including the difficulty of controlling the chain length through condensation reactions and the insolubility of the polymer when more that four or five phenylrings are linked together. A more serious problem is the lack of coplanarity of the phenyl rings due to the steric interactions between the hydrogen atoms in the ortho positions. This raises the band gap and, in applications such as light-emitting diodes (see Chapter 14), limits the emission color to the blue region of the spectrum.
4. Polypyrrole and Polythiophene
Pyrrole, thiophene, and their substituted derivatives can be polymerized electrochemically as shown in reactions 7 and 8. The polymers form as films on the cathode, and can even be produced as a continuous strip by use of a revolving, metal drum electrode. Undoped polypyrrole and polythiophene have very low conductivities, in the region of 10−12S/cm. When doped chemically or electrochemically, the conductivity rises to the 102 or 103S/cm level, which is in the same range as silver. Polypyrrole is a black material that has been considered for use as an electrode component in batteries, while polythiophene is of interest for its ability to change color in the presence of different compounds, which makes it potentially useful as a sensor.
5. Polyaniline
This is a polymer that has been known almost from the beginning of organic chemistry, although not recognized as such. It is formed by the oxidation of aniline either chemically (using ammonium peroxydisulfate) or electrochemically (reaction 9). The oxidation process goes through several stages, each of which gives rise to a different level of conductivity. The polymer structure shown in reaction 9, where x + y = 1, is a good starting point for understanding the different forms of this polymer. Leucoemeraldine, with x = 1 and y = 0, is the least oxidized form, whereas pernigraniline, with x = 0 and y = 1, is the most oxidized state. The most useful form for practical applications is the green-colored “emeraldine base” state where both x and y = 0.5, which is a semiconductor when doped with an acid such as HCl.
Emeraldine base is the most useful form because it is less prone to degradation in the atmosphere than the other two modifications. Moreover, it can be synthesized in the form of nanofibers, is used for corrosion protection and antistatic coatings, and is employed as the thin, hole-injection layer in light-emitting diodes. A demonstration integrated circuit has been built based on polyaniline transistors imaged by photolithography. The imaging process is based on the fact that irradiation of the polymer by strong ultraviolet light reduces the conductivity by a factor of 10.
6. Mechanism of Conduction in Unsaturated Organic Polymers
Although it was originally suspected that semiconduction in doped organic unsaturated polymers might occur via a similar mechanism to that found in doped silicon, it was soon realized that significant differences existed. First, much larger amounts of dopant are needed than in conventional inorganic semiconductors, and the dopants are oxidizing or reducing molecules rather than atoms. Further, attempts to detect significant amounts of unpaired electrons in the doped polymers by electron spin resonance spectroscopy were unsuccessful. Hence it appeared that conduction may not occur simply via free electrons moving down the π-congugated backbone (Figure 10.5a). It was also noted that the polymer chains are shorter than the length of the conductive sample, and so the question arose as to how electrons, if they are the charge carriers, jump from the end of one polymer chain to another.
The initial theory was based on polyacetylene conduction. The most widely accepted explanation is that pure polyacetylene is a wide-bandgap semiconductor (Figure 10.5b). However, the pristine polymer shows evidence of an e.s.r. signal that would be consistent with unpaired electrons (Figure 10.5a). The addition of an electron to a conjugated polymer chain initially generates a radical anion, called a polaron, in which three electrons occupy two energy levels within the bandgap. Addition of a second electron yields a dianion or bipolaron, which is unstable because of the proximity of the two negative charges. Thus, the two electron pairs migrate away from each other to a safe distance that equalizes their energies in the band gap (a soliton pair), and conduction occurs as these two electron pairs migrate in tandem along the polymer chain or transfer from chain to chain. From a chemist’s perspective it would be expected that the movement of a soliton or bipolaron along an unsaturated polymer chain would require a lengthening and shortening of skeletal bonds as the charge carrying unit moves through each site on the polymer—a relatively slow process. This is the same problem that has been used to argue against conduction by isolated electrons (solitons). Removal of an electron from a polymer would generate a radical cation, and similar arguments apply. It appears that hole conduction via radical cations may be more important in unsaturated polymers than conduction by electrons or dianions. Explanations for electronic conduction in polyacetylene and the other conductive polymers are still evolving.
E. PHOTOLITHOGRAPHY AND MICROLITHOGRAPHY
1. Principles of Semiconductor Fabrication
Photolithography is based on an old method for printing photographs known variously as the dye transfer process or the “washoff relief process.” In this process (no longer employed for everyday photography) a sheet of transparent film is coated with a thin layer of a photocrosslinkable polymer that was often gelatin containing dichromate as a sensitizer. Light passing through a negative to the sensitized film caused crosslinking of the gelatin, but only in those areas exposed to light. After a development process to amplify the crosslinking, the unexposed, uncrosslinked gelatin was removed by washing with warm water to leave a raised relief image that corresponded to the transparent parts of the negative. This raised relief image was then allowed to absorb a water-soluble dye, and the printing “plate” or matrix was pressed in contact with a sheet of paper to allow the dye to diffuse to the paper in proportion to the thickness of the relief image. Thus, a single printing plate could be used to make many prints by repeating the dye absorption–printing cycle. Full-color prints were produced by superimposing dyes from three separate matrices to transfer yellow, magenta, and cyan colors to the print.
Figure 10.5. Electronic conduction in a polymeric semiconductor such as polyacetylene, showing the formation of a conductive soliton pair.

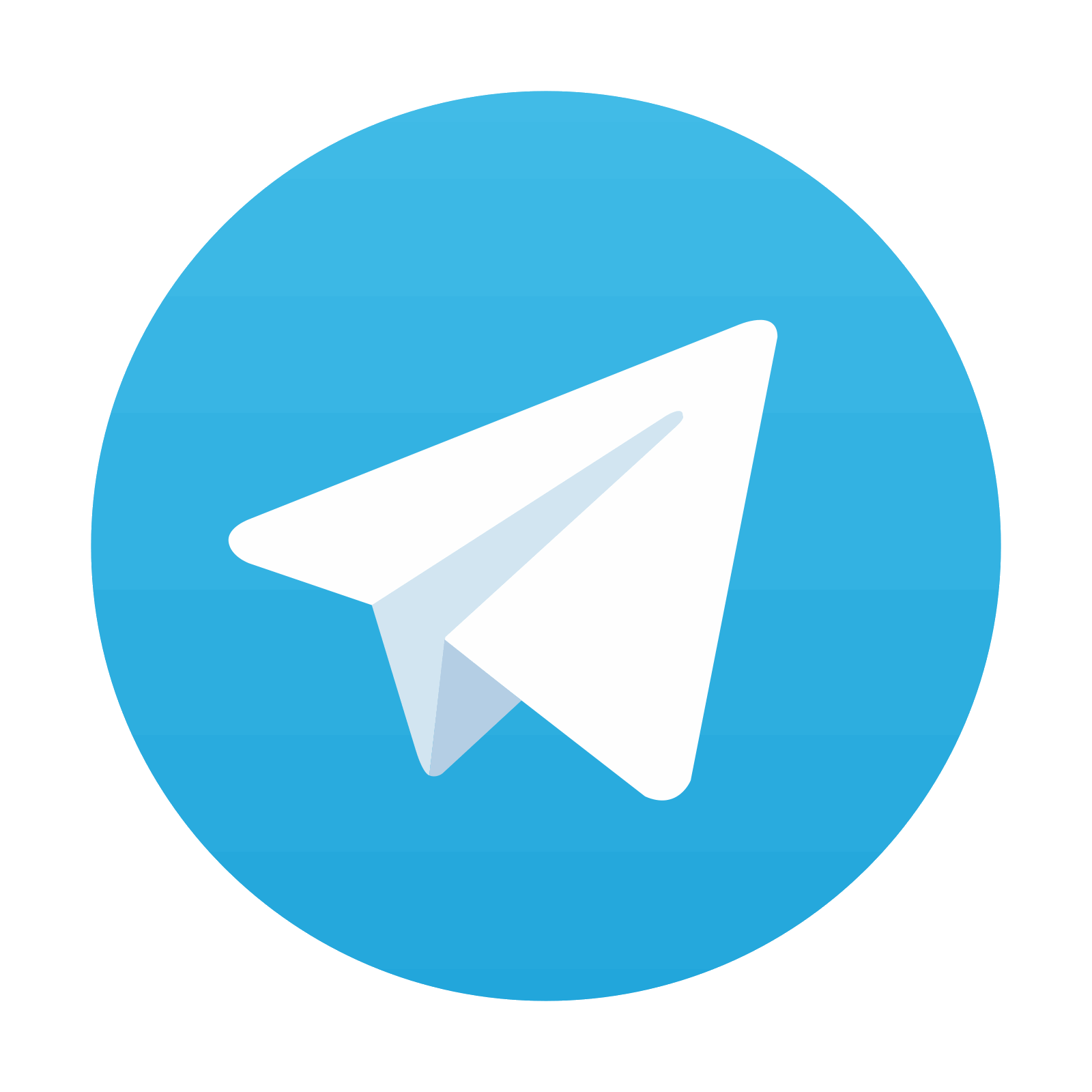
Stay updated, free articles. Join our Telegram channel
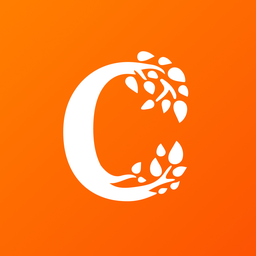
Full access? Get Clinical Tree
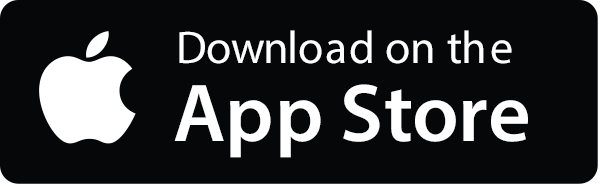
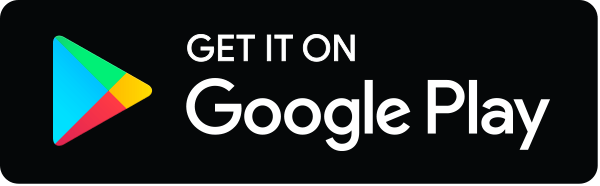