Selenium (Se) was discovered in 1817 by the Swedish chemist Jöns Jakob Berzelius, who found the element associated with tellurium. He named the new element selenium (from the Greek word selene, meaning moon) because it tends to be found in the earth along with tellurium (which had been named after the Latin word tellus, meaning earth). Not until the late 1950s, however, was selenium thought to play a role in normal metabolism. Until that time, the biomedical significance of selenium had been recognized only for its toxic properties. In 1957 it was discovered that trace amounts of Se could alleviate necrotic liver disease and capillary leakage in vitamin E–deficient animals, suggesting that Se spared the need for that fat-soluble vitamin (Schwarz and Foltz, 1957; Schwarz et al., 1957). Research in the 1970s revealed the basis of this interaction: Se is an essential constituent of the antioxidant enzyme glutathione peroxidase 1 (GPX1) (Rotruck et al., 1973). Since that time, an increasing understanding has emerged of the metabolic functions and health implications of this trace element, now known to be an essential constituent of some 25 selenoproteins, including the GPXs, each of which contains covalently linked selenium in the form of selenocysteine (SeCys). This previously unrecognized selenoamino acid, SeCys, was found to be incorporated into the selenoproteins cotranslationally by a process signaled by the TGA codon in DNA (UGA in mRNA), which normally functions as a stop codon. Because many, if not all, selenoproteins appear to have redox functions, selenium is now regarded as being important in the metabolic protection from cellular oxidative stress. In addition, selenium has been shown to have a role in anticarcinogenesis. The major forms of selenium in cells are the selenoamino acids, in which selenium forms covalent bonds with carbon atoms. Selenium also forms covalent bonds with sulfur atoms. The selenoamino acids include SeCys, which is encoded in a special manner by DNA/mRNA and is cotranslationally synthesized, and the selenide-containing amino acid selenomethionine (SeMet, a selenoether), which originates in plants and microorganisms that use elemental selenium in place of sulfur for synthesis of sulfur (seleno) amino acids. SeMet can be incorporated into proteins in the body nonspecifically in place of methionine (Met). The selenium compounds of greatest relevance in biology are listed in Table 39-1. TABLE 39-1 Biologically Important Se Compounds Foods of all types tend to show geographic patterns of variation in selenium content reflecting, in general, local soil selenium conditions. Variation in the selenium content of foods is readily seen by comparing the selenium contents of like foods from different countries (Combs and Combs, 1986). For example, whole wheat grain may contain more than 2 to 5 mg Se/kg (air-dried) if produced in the western parts of North and South Dakota in the United States or in Saskatchewan and Alberta in Canada. However, whole wheat grain may contain as little as 0.1 mg Se/kg if produced in Kansas or New Zealand, and only 0.005 mg Se/kg if produced in Shaanxi Province, China. On a global basis, foods with the lowest selenium contents are found in the provinces of Heilongjiang, northern Shaanxi, and Sichuan in China. Ironically, foods containing the greatest concentrations of selenium have also been found in China, though in different locales. Most of the selenium taken up by plants is incorporated into organoselenium compounds such as SeMet, Se-methylselenocysteine, SeCys, and other related metabolites (Rayman et al., 2008). The selenoamino acids are synthesized by plants and microorganisms when Se2− replaces S2− in the biosynthetic pathways for sulfur-amino acid synthesis. The selenoamino acids, particularly SeMet, then are incorporated into proteins. SeMet is the most abundant form of selenium in cereal grains and legumes, due mainly to the nonspecificity of the methionyl-tRNA synthetase which will use SeMet in place of Met for aminoacylation of the tRNAMet. sources of selenium means that, in many countries, selenium intakes can be affected by factors that influence the importation of grain from the world market, most of which is grown in areas of the United States, Canada, and Australia where the soil is rich in selenium. An analysis of American diets based on the U.S. Department of Agriculture 1977–1978 Nationwide Food Consumption Survey and published selenium contents of American foods revealed that a core of only 22 foods provided 80% of the total dietary selenium intake (Schubert et al., 1987). Five foods contributed half of total selenium in the “typical” American diet; these foods were beef, white bread, pork, chicken, and eggs. In general, the apparent absorption of selenium from food is efficient. Inorganic Se salts appear to be absorbed across the gut by diffusion, whereas SeMet is actively transported by the same system that transports methionine (Met). Accordingly, the enteric absorption of relatively large single doses (200 μg Se) was estimated to be 84% for selenite and 98% for SeMet (Patterson et al., 1989). The majority of selenium appears to be taken up by liver to reenter the circulation as selenoprotein P (SepP), which is essential for the normal distribution of selenium to extrahepatic tissues (Burk and Hill, 2009). Recognition that the metabolic function of selenium is related to that of vitamin E (α-tocopherol) emerged with the demonstration that selenium could prevent pathological conditions in vitamin E–deficient rats (Schwarz and Foltz, 1957) and chicks (Schwarz et al., 1957). From this origin came the view of selenium as an antioxidant nutrient. Indeed, combined deprivation of selenium and vitamin E yields elevated levels of products of free radical attack on polyunsaturated fatty acids that are detectable in tissues (malonyldialdehyde, F2-isoprostanes) and exhaled breath (ethane, pentane). Thus it is clear that selenium and vitamin E function in concert in cellular antioxidant protection. In this system, α-tocopherol functions as a lipid-soluble, chain-breaking antioxidant, whereas selenium is involved as one or more SeCys residues in one or more redox-active selenoproteins. Because the physiological role of selenium is as SeCys residues in selenoproteins, an important aspect of selenium metabolism is the incorporation of dietary selenium into these selenoproteins. The various forms of selenium that are taken up from the gastrointestinal tract are metabolized ultimately to selenide (Ganther, 1999) (Figure 39-1). For the oxidized inorganic forms, this involves reduction. Selenate is converted to selenite via activation to adenosine phosphoselenate and reduction by reactions with reduced glutathione (GSH). Selenite, whether from the diet or formed from selenate, is converted to selenide by reactions that require GSH and NADPH as reductants and involve formation of a glutathione selenopersulfide (GSSeH) intermediate. Ingested SeMet can be used in protein synthesis in place of Met, or it can be catabolized ultimately to selenide. It can charge tRNAMet at rates similar to that of Met (Km values: 11 μmol/L for SeMet versus 7 μmol/L for Met) and is thus readily incorporated into proteins according to their Met contents. This nonspecific, protein-bound selenium accounts for most of the selenium in tissues (e.g., 20% to 60% of the selenium in plasma) of individuals fed normal diets containing SeMet. Metabolism of SeMet to selenide occurs by the same pathway used for the transmethylation and transsulfuration of Met to form Cys. This involves activation of SeMet to Se-adenosylselenomethionine, which in turn serves as a methyl donor and is converted to Se-adenosylselenohomocysteine. Se-adenosylselenohomocysteine is further metabolized to selenohomocysteine. Selenohomocysteine is further metabolized by the transsulfuration enzymes (see Figure 14-22 for Met metabolism Chapter 14), cystathionine β-synthase and cystathionine γ-lyase. These reactions yield SeCys, from which selenide is released by a specific γ-lyase. The SeCys produced from metabolism of ingested SeMet is not incorporated directly (i.e., nonspecifically) into proteins in animals. A third prospect for SeMet is metabolism to methylated forms and methylseleno-N-acetyl-galactosamine, which are excretable forms. The circulating plasma pool of selenium comprises two functional, specific selenoproteins (SepP1 and GPX3); the general non-specific SeMet in albumin and other plasma proteins; and a small amount of selenium in forms other than amino acid residues in proteins (e.g., selenosugars). SepP1 and GXP3 accounted for about 34% and 20%, respectively, (or a total of 54%) of the total plasma selenium pool in a group of healthy adult subjects in North Dakota in the United States, a region with relatively high selenium intakes and a mean plasma selenium level of 142 ng/mL (Combs et al., 2011). The proportion of plasma selenium contributed by the two plasma selenoproteins was estimated to be slightly larger (64%) in a group of subjects with plasma total selenium concentrations of 125 ng/mL, which are closer to mean values for the U.S. population (Burk et al., 2006). Maximal expression of the plasma selenoproteins occurs at intakes yielding plasma levels above 70 ng/mL, which is close to the plasma selenium levels estimated to be contributed by maximal expression of SepP1 and GPX3. Higher intakes of foods containing SeMet result in increases in the nonspecific incorporation of SeMet into plasma proteins and thus higher plasma selenium levels. Intake of inorganic forms of selenium does not result in an increase in this component of plasma selenium because animals do not incorporate inorganic selenium into SeMet (Burke et al., 2001). Metabolic tracer studies have shown that over a 12-day period, Se-adequate adults excrete a total of 17% of selenium from an oral selenite dose, but only 11% of selenium from an oral SeMet dose (Patterson et al., 1989). The primary route of selenium excretion is across the kidney; the dominant urinary metabolite in individuals with low-to-moderate Se intakes is the selenosugar 1β-methylseleno-N-acetyl-galactosamine (Kobayashi et al., 2002). Individuals with relatively high selenium intakes may excrete other compounds such as trimethylselenonium or other selenosugars in the urine and methylselenol in the breath. Selenium is incorporated as SeCys in some 25 selenoproteins in animals (Kryukov et al., 2003). Neither SeCys from the diet or endogenous protein turnover nor SeCys formed in the body during SeMet catabolism can be used directly for the specific incorporation of SeCys into selenoproteins. Instead, inorganic selenide is activated and added in a cotranslational conversion of serine (Ser) to SeCys while the amino acid is bound to a specific tRNA (tRNA[Ser]SeCys) (Figure 39-2). The selenium in selenoamino acids is used for selenoprotein synthesis only after the selenoamino acids have been catabolized to yield inorganic selenium. The tRNA[Ser]SeCys in mammals differs from other tRNAs by having 90 nucleotides (compared to the usual 76), a UCA (uracil–cytosine–adenine) anticodon sequence, 2 additional base pairs in the acceptor stem (9 compared to 7), and 11 additional bases (16 compared to 5, including 5 base pairs compared to none) in the D-loop (Carlson et al., 2006). Mammalian tRNA[Ser]SeCys has two isoforms arising from the same gene and differing in the modification of uridine at the 5′ position of the UCA anticodon (i.e., 5-methylcarboxymethyl uridine or 5-methylcarboxymethyl uridine-2′-O-methylribose). The tRNA[Ser]SeCys is charged with Ser at its 3′-terminal adenosine in a reaction catalyzed by seryl-tRNA synthetases, which do not distinguish between tRNASer and tRNA[Ser]SeCys. Selenophosphate is the immediate Se donor for the conversion of Ser-tRNA[Ser]SeCys to SeCys-tRNA[Ser]SeCys. The formation of selenophosphate is catalyzed by selenophosphate synthetase (SPS) (Salinas et al., 2006). Humans have two SPS genes; one encodes a selenoprotein (SPS2) and the other encodes a non-selenoprotein (SPS1). SPS2 and SPS1 differ only in the amino acid residue at position 60 (threonine in SPS1, SeCys in SPS2). It is hypothesized that SPS1 may ensure continued synthesis of SeCys, albeit at low levels, under conditions of limited Se supply and diminished activity of SPS2. The reaction uses ATP as a cosubstrate for addition of phosphate to selenide (HSe2–) to form selenophosphate (HSePO32−). Each SeCys center of a selenoprotein is encoded by a TGA in the respective gene, which is transcribed as UGA in the mRNA. This phenomenon, first recognized with the sequencing of the cDNA for GPX1, was unexpected because a UGA in mRNAs typically serves as a stop codon in mammalian protein synthesis. Studies on translation of selenoprotein mRNAs revealed that the 3′-untranslated region (3′-UTR) of the mRNA was necessary for UGA-encoded SeCys incorporation. Berry and colleagues (1991) identified a consensus 87-base stem–loop element necessary for the insertion of Se in two selenoproteins of the rat (iodothyronine 5′-deiodinase-1 and GPX1). This SeCys insertion sequence (SECIS) element has been found in the 3′-UTRs of the mRNAs of all selenoproteins. Most selenoprotein mRNAs contain a single UGA codon encoding a single SeCys residue per polypeptide chain and a single SECIS element. Selenoprotein P is unique in that its mRNA encodes multiple SeCys residues and contains two SECIS elements in its 3′-UTR. As shown in Figure 39-3, the mammalian SECIS element consists of a stem–loop structure with an apical loop and an internal loop between two helical regions (stems). The apical loop consists of 7 to 10 unpaired bases, including a consensus AA (adenine–adenine) sequence. The helix between the apical and the internal loops contains an AUGA (adenine–uracil–guanine–adenine) sequence 5′ to the apical loop, and a GA (guanine–adenine) sequence 3′ to the apical loop. This AUGA … AA … GA motif is key to functionality, although a few mammalian SECIS elements have been found with a variant motif, AUGA … CC … GA. The conserved UGA and GA sequences form the core of a quartet motif of non–Watson and Crick base pairs (i.e., U-U and G-A), resulting in a greater than 90-degree kink in the stem–loop. Half of mammalian SECIS elements have an additional stem extending from the apical loop; it is thought that these specific secondary structures may affect the rate of SeCys insertion into various selenoproteins. Archaea have the same SECIS element as eukaryotes. However, the prokaryotic SECIS consists of a 38-nucleotide stem–loop element located in the open reading frame of the selenoprotein mRNA immediately following the UGA codon (Bock et al., 2006). It is thought that constraints of maintaining this secondary structure may limit the capacity of bacterial genes to place SeCys at the active site of enzymes. In bacteria, a single elongation factor is required to bind the SECIS element and the SeCys-tRNA, whereas in eukaryotes and archaea, those roles are filled by different proteins: SECIS-binding protein (SBP2), which appears to bind to the AUGA/GA stem nucleotides, and a SeCys-specific elongation factor (eEFSeCys) that partners with SBP2 for incorporation of UGA-encoded SeCys. That eEFSeCys binds to SeCys-tRNA[Ser]SeCys but not to Ser-tRNASer prevents misincorporation of Ser in place of SeCys. Like other elongation factors, eEFSeCys binds GTP and is dependent upon GTP hydrolysis for activity. Genomic screening for selenoproteins has been accomplished by searching for conserved SECIS elements, conserved appropriate secondary structures, open reading frames containing in-frame TGA codons, and homologs in other species containing Cys (Kryukov et al., 2003). Most selenoproteins appear to be enzymes, or to have been derived from enzyme families, with their SeCys residues located in variants of the CxxC (where C = Cys and x = any amino acid) motif found in many redox proteins (Gladyshev, 2006). The selenoproteins appear to have a scattered phylogenetic distribution; most have homologs containing Cys instead of SeCys. For example, GPX6 is a selenoprotein (contains SeCys) in humans and swine but not in rodents. Other cross-species examples include methionine-R-sulfoxide reductase, which is a selenoprotein in green algae but not in vertebrates; and protein U, which contains SeCys in fish but exists as Cys-containing homologs in humans and worms. In general, the substitution of SeCys for Cys in the enzyme active site enhances its catalytic efficiency. Selenoproteins are present in bacteria, archaea, and eukaryota, but not in all members of these domains. In addition, the size of the selenoproteome varies among domain members. For example, in eukaryotes, the highest number of selenoproteins is observed in aquatic organisms (e.g., more than 30 selenoproteins in many fishes and algae) whereas no selenoproteins have been identified in fungi and higher plants (Lobanov et al., 2009). In addition to use of selenium for synthesis of proteins that are translated with SeCys residues, some bacterial and archaeal cells use selenium for synthesis of selenouridine-modified tRNAs and/or posttranslational maturation of selenium-molybdenum-cofactor containing enzymes such as xanthine dehydrogenase and purine hydroxylase (Haft and Self, 2008). The incorporation of selenium into these latter compounds also appears to require the conversion of selenide to selenophosphate by selenophosphate synthetase. The human selenoproteome consists of 25 selenoproteins broadly classified as antioxidant enzymes (Reeves and Hoffman, 2009) (Table 39-2). Of these, the first to be recognized, and thus the best studied, are the GPXs. Other well-studied selenoproteins include the iodothyronine 5′-deiodinases (DIs), the thioredoxin reductases (TRRs), and selenoproteins P (SepP) and W (SepW). Many selenoenzymes exist as multiple isoforms. TABLE 39-2
Selenium
Chemistry of Selenium
OXIDATION STATE
COMPOUND
BIOLOGICAL RELEVANCE
Se2–
Hydrogen selenide, H2Se
Methylselenol, CH3SeH
Dimethyl selenide, (CH3)2Se
Trimethyl selenonium, (CH3)3Se+
1β-Methylseleno-N-acetyl-D-galactosamine
Selenomethionine, SeMet
Selenocysteine, SeCys
Se-Methylselenomethionine
Se-Methylselenocysteine
Selenobetaine
Selenotaurine
Obligate metabolic precursor to selenoproteins
Excretory form (lung); putative anticarcinogenic metabolite
Excretory form (lung)
Excretory form (kidney)
Excretory form (kidney)
Common food form, non-specific Se-containing proteins (e.g., albumin); metabolized to selenocysteine (SeCys)
Common food form; form in selenoproteins; metabolized to hydrogen selenide
Form in some foods; metabolized to methylselenol
Form in some foods; metabolized to methylselenol
Metabolic precursor of methylselenol
Form in some foods
Se0
Selenodiglutathione
Reductive metabolite of selenite/selenate with anticarcinogenic activity
Se4+
Sodium selenite, Na2SeO3
Commonly used supplement for livestock feeds and ingredient in multivitamin/mineral supplements
Se6+
Sodium selenate, Na2SeO4
Potential food/feed supplement form
Selenium in Foods
Selenium in Human Diets
Utilization of Dietary Selenium
Bioavailability of Dietary Selenium
Selenium Absorption
Selenium Metabolism
Reduction of Selenate and Selenite to Selenide
Metabolism of Selenomethionine from the Diet
Plasma Forms of Selenium
Selenium Excretion
Selenium Incorporation into Selenoproteins
tRNA[Ser]SeCys
The UGA Codon in Selenoprotein mRNA
The Selenoproteins
SELENOPROTEIN
GENERAL FUNCTION
ISOFORMS
LOCALIZATION
SPECIAL FEATURES
Glutathione peroxidases
Antioxidant enzymes catalyzing the reduction of hydroperoxides using reducing equivalents from reduced glutathione (GSH)
GPX1
Cytosol, mitochondrial matrix space
Sensitive to dietary Se deprivation
GPX2
Cytosol; mostly gastrointestinal cells
Relatively resistant to dietary Se deprivation
GPX3
Plasma, milk
Sensitive to dietary Se deprivation
GPX4
Cell membranes; mammalian sperm mid-piece
Relatively resistant to dietary Se deprivation
Thioredoxin reductases
Antioxidant flavoenzymes catalyzing the reduction of thioredoxin using reducing equivalents from NADPH
TRR1
Cytosol
TRR2
Mitochondria
Relatively resistant to dietary Se deprivation
TRR3
Cytosol; mostly testes
Deiodinases
Enzymes essential for thyroid hormone function; catalyze activation of T4 to T3
DI1
Cytosol; mostly liver, muscle
Catalyzes 5′-deiodination of T4 outer ring; also can catalyze 5-deiodination of inner ring
DI2
Cytosol; brain, pituitary, brown adipose, skin, placenta
Catalyzes 5′-deiodination of T4 outer ring
DI3
Cytosol; brain, skin, placenta
Catalyzes 5-deiodination of inner ring for conversion of T3 to T2 and T4 to reverseT3
Selenoprotein P
Major transporter for Se to peripheral tissues
SepP
Plasma; cytosol of most cells
Has 5–6 Se atoms per molecule
Selenoprotein W
Presumed to function in protecting muscle from oxidative damage
SepW
Cytosol; muscle, brain
Upregulated by supranutritional Se levels
Selenoprotein 15
Thought to be involved in protein folding
Sep15
Endoplasmic reticulum; most cells
A thiol-disulfide oxidase
Selenoprotein R
Reduces methionine R-sulfoxide (oxidized methionine)
SepR
Cytosol and nucleus; most cells
Also called methionine-R-sulfoxide reductase
Selenophosphate synthetase 2
Catalyzes ATP-dependent activation of selenide in the cotranslational synthesis of tRNA-bound SeCys
SPS2
Cytosol; most cells
Functions in the synthesis of all selenoproteins Stay updated, free articles. Join our Telegram channel
Full access? Get Clinical Tree
Selenium
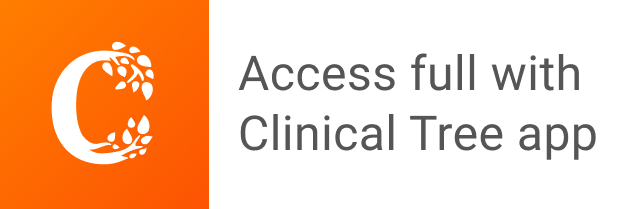