CHAPTER 36 Role of the Kidneys in the Regulation of Acid-Base Balance
The concentration of H+ in body fluids is low in comparison to the concentration of other ions. For example, Na+ is present at a concentration some 3 million times greater than that of H+ ([Na+] = 140 mEq/L; [H+] = 40 nEq/L). Because of the low [H+] of body fluids, it is commonly expressed as the negative logarithm, or pH.
Virtually all cellular, tissue, and organ processes are sensitive to pH. Indeed, life cannot exist outside a range of body fluid pH from 6.8 to 7.8 (160 to 16 nEq/L of H+). Normally, the pH of extracellular fluid (ECF) is maintained between 7.35 and 7.45. As described in Chapter 2, the pH of intracellular fluid (ICF) is slightly lower (7.1 to 7.2), but also tightly regulated.
THE HCO3− BUFFER SYSTEM
As indicated, the first reaction (hydration/dehydration of CO2) is the rate-limiting step. This normally slow reaction is greatly accelerated in the presence of carbonic anhydrase.* The second reaction, the ionization of H2CO3 to H+ and HCO3− is virtually instantaneous.
The Henderson-Hasselbalch equation (36-2) is used to quantitate how changes in CO2 and HCO3− affect pH.
or
In these equations, the amount of CO2 is determined from the partial pressure of CO2 (PCO2) and its solubility (α) in solution. For plasma at 37° C, α has a value of 0.03. Also, pK′ is the negative logarithm of the overall dissociation constant for the reaction in Equation 36-1 and has a value of 6.1 for plasma at 37° C. Alternatively, the relationship between HCO3−, CO2, and [H+] can be expressed as follows:
Inspection of Equations 36-3 and 36-4 show that pH and [H+] vary when either [HCO3−] or PCO2 is altered. Disturbances in acid-base balance that result from a change in [HCO3−] are termed metabolic acid-base disorders, whereas those resulting from a change in PCO2 are termed respiratory acid-base disorders. These disorders are considered in more detail in a subsequent section. The kidneys are primarily responsible for regulating [HCO3−] in ECF, whereas the lungs control PCO2.
OVERVIEW OF ACID-BASE BALANCE
The diet of humans contains many constituents that are either acid or alkali. In addition, cellular metabolism produces acid and alkali. Finally, alkali is normally lost each day in feces. As described later, the net effect of these processes is the addition of acid to body fluids. For acid-base balance to be maintained, acid must be excreted from the body at a rate equivalent to its addition. If addition of acid exceeds excretion, acidosis results. Conversely, if excretion of acid exceeds addition, alkalosis results.
The major constituents of the diet are carbohydrates and fats. When tissue perfusion is adequate, O2 is available to tissues, and insulin is present at normal levels, carbohydrates and fats are metabolized to CO2 and H2O. On a daily basis, 15 to 20 mol of CO2 is generated through this process. Normally, this large quantity of CO2 is effectively eliminated from the body by the lungs. Therefore, this metabolically derived CO2 has no impact on acid-base balance. CO2 is usually termed volatile acid because it has the potential to generate H+ after hydration with H2O (Equation 36-1). Acid not derived directly from the hydration of CO2 is termed nonvolatile acid (e.g., lactic acid).
NET ACID EXCRETION BY THE KIDNEYS
The kidneys cannot excrete urine more acidic than pH 4.0 to 4.5. Even at a pH of 4.0 only 0.1 mEq/L of H+ can be excreted. Therefore, to excrete sufficient acid, the kidneys excrete H+ with urinary buffers such as phosphate (Pi).* Other constituents of urine can also serve as buffers (e.g., creatinine), although their role is less important than that of Pi. Collectively, the various urinary buffers are termed titratable acids. This term is derived from the method by which these buffers are quantitated in the laboratory. Typically, alkali (OH−) is added to a urine sample to titrate its pH to that of plasma (i.e., 7.4). The amount of alkali added is equal to the amount of H+ titrated by these urine buffers and is termed titratable acid.
where (UNH4+ × ) and (UTA ×
) are the rates of excretion (mEq/day) of NH4+ and titratable acid (TA) and (UHCO3− ×
) is the amount of HCO3− lost in urine (equivalent to adding H+ to the body).* Again, maintenance of acid-base balance means that net acid excretion must equal nonvolatile acid production. Under most conditions, very little HCO3− is excreted in urine. Thus, net acid excretion essentially reflects titratable acid and NH4+ excretion. Quantitatively, titratable acid accounts for approximately a third and NH4+ for two thirds of net acid excretion.
HCO3− Reabsorption along the Nephron
As indicated by Equation 36-7, net acid excretion is maximized when little or no HCO3− is excreted in urine. Indeed, under most circumstances, very little HCO3− appears in urine. Because HCO3− is freely filtered at the glomerulus, approximately 4320 mEq/day is delivered to the nephrons and then reabsorbed. Figure 36-1 summarizes the contribution of each nephron segment to reabsorption of the filtered HCO3−.
The proximal tubule reabsorbs the largest portion of the filtered load of HCO3−. Figure 36-2 summarizes the primary transport processes involved. H+ secretion across the apical membrane of the cell occurs by both an Na+-H+ antiporter and H+-ATPase. The Na+-H+ antiporter (NHE3) is the predominant pathway for H+ secretion and uses the lumen-to-cell [Na+] gradient to drive this process (i.e., secondary active secretion of H+). Within the cell, H+ and HCO3− are produced in a reaction catalyzed by carbonic anhydrase. The H+ is secreted into tubular fluid, whereas HCO3− exits the cell across the basolateral membrane and returns to the peritubular blood. Movement of HCO3− out of the cell across the basolateral membrane is coupled to other ions. The majority of HCO3− exits via a symporter that couples the efflux of 1Na+ with 3HCO3− (sodium bicarbonate cotransporter: NBC1). In addition, some of the HCO3− may exit in exchange for Cl− (via Na+-independent and/or Na+-dependent Cl−-HCO3− antiporters). As noted in Figure 36-2, carbonic anhydrase is also present in the brush border of the proximal tubule cells. This enzyme catalyzes the dehydration of H2CO3 in luminal fluid and thereby facilitates reabsorption of HCO3−.
Carbonic anhydrases are zinc-containing enzymes that catalyze the hydration of CO2 (see Equation 36-1). The isoform CA-I is found in red blood cells and is critical for these cells’ ability to carry CO2. Two isoforms, CA-II and CA-IV, play important roles in urine acidification. The CA-II isoform is localized to the cytoplasm of many cells along the nephron, including the proximal tubule, thick ascending limb of Henle’s loop, and intercalated cells of the distal tubule and collecting duct. The CA-IV isoform is membrane bound and exposed to the contents of the tubular fluid. It is found in the apical membrane of both the proximal tubule and thick ascending limb of Henle’s loop, where it facilitates reabsorption of the large amount of HCO3− reabsorbed by these segments. CA-IV has also been demonstrated in the basolateral membrane of the proximal tubule and thick ascending limb of Henle’s loop. Its function at this site is thought to facilitate the exit of HCO3− from the cell in some way.
The distal tubule* and collecting duct reabsorb the small amount of HCO3− that escapes reabsorption by the proximal tubule and loop of Henle. Figure 36-3 shows the cellular mechanism of H+/HCO3− transport by intercalated cells located within these segments (see Chapter 32).
A second population of intercalated cells secrete HCO3− rather than H+ into the tubular fluid (also called B- or β-intercalated cells).† In these cells, the H+-ATPase is located in the basolateral membrane, and the Cl−-HCO3− antiporter is located in the apical membrane (Fig. 36-3). However, the apical membrane Cl−-HCO3− antiporter is different from the one found in the basolateral membrane of the H+-secreting intercalated cells and has been identified as pendrin. Other HCO3− transporters have been localized to the HCO3−-secreting intercalated cell, but their precise role in the function of the cell has not been defined. The activity of the HCO3−-secreting intercalated cell is increased during metabolic alkalosis, when the kidneys must excrete excess HCO3−. However, under most conditions (i.e., ingestion of a meat-containing diet), H+ secretion predominates in these segments.
The apical membrane of collecting duct cells is not very permeable to H+, and thus the pH of tubular fluid can become quite acidic. Indeed, the most acidic tubular fluid along the nephron (pH of 4.0 to 4.5) is produced there. In comparison, the permeability of the proximal tubule to H+ and HCO3− is much higher, and tubular fluid pH falls to only 6.5 in this segment. As explained later, the ability of the collecting duct to lower the pH of tubular fluid is critically important for the excretion of urinary titratable acids and NH4+.
Regulation of H+ Secretion
A number of factors regulate secretion of H+ and thus reabsorption of HCO3− by cells of the nephron (Table 36-1). From a physiological perspective, the primary factor that regulates H+ secretion by the nephron is a change in systemic acid-base balance. Thus, acidosis stimulates H+ secretion, whereas H+ secretion is reduced during alkalosis. The response of the kidneys to changes in acid-base balance includes both immediate changes in the activity or number of transporters in the membrane (or both) and longer-term changes in the synthesis of transporters. For example, with metabolic acidosis, whether produced by a decrease in ECF [HCO3−] or by an increase in the partial pressure of carbon dioxide (PCO2), the pH of cells of the nephron decreases. This will stimulate H+ secretion by multiple mechanisms, depending on the particular nephron segment. First, the decrease in intracellular pH will create a more favorable cell-to–tubular fluid [H+] gradient and thereby make the secretion of H+ across the apical membrane more energetically favorable. Second, the decrease in pH may lead to allosteric changes in transport proteins, thereby altering their kinetics. This has been reported for the Na+-H+ antiporter (NHE3) in the proximal tubule. Finally, transporters may be shuttled to the membrane from intracellular vesicles. This mechanism occurs in both the intercalated cells of the collecting duct, where acidosis stimulates the exocytotic insertion of H+-ATPase into the apical membrane, and in the proximal tubule, where insertion of the Na+-H+ antiporter and H+-ATPase into the apical membrane occurs. With long-term acidosis, the abundance of transporters increases, either by increased transcription of appropriate transporter genes or by increased translation of transporter mRNA. Examples include the Na+-H+ antiporter and the 1Na+-3HCO3− symporter in the proximal tubule and H+-ATPase in the intercalated cell.
Table 36-1 Factors Regulating H+ Secretion (HCO3− Reabsorption) by the Nephron
Factor | Primary Site of Action |
---|---|
Increased H+ Secretion | |
Primary | |
Decrease in ECF [HCO3−] (↓pH) | Entire nephron |
Increase in arterial PCO2 | Entire nephron |
Cortisol | Proximal tubule* |
Endothelin | Proximal tubule* |
Secondary | |
Increase in the filtered load of HCO3− | Proximal tubule |
ECF volume contraction | Proximal tubule |
Angiotensin II | Proximal and distal tubules |
Aldosterone | Distal tubule and collecting duct |
Hypokalemia | Proximal tubule |
PTH (chronic) | Thick ascending limb; distal tubule |
Decreased H+ Secretion | |
Primary | |
Increase in ECF [HCO3−] (↑pH) | Entire nephron |
Decrease in arterial PCO2 | Entire nephron |
Secondary | |
Decrease in the filtered load of HCO3− | Proximal tubule |
ECF volume expansion | Proximal tubule |
Hypoaldosteronism | Distal tubule and collecting duct |
Hyperkalemia | Proximal tubule |
PTH (acute) | Proximal tubule |
* Effect on the proximal tubule is established. It may also regulate H+ secretion in other nephron segments.
Table 36-1 also lists other factors that influence secretion of H+ by cells of the nephron. However, these factors are not directly related to the maintenance of acid-base balance. Because H+ secretion in the proximal tubule and thick ascending limb of the loop of Henle is linked to the reabsorption of Na+ (via the Na+-H+ antiporter), factors that alter Na+ reabsorption secondarily affect H+ secretion. For example, the process of glomerulotubular balance ensures that the reabsorption rate of the proximal tubule is matched to the glomerular filtration rate (GFR) (see Chapter 33). Thus, when the GFR is increased, the filtered load to the proximal tubule is increased, and more fluid (including HCO3−) is reabsorbed. Conversely, a decrease in the filtered load results in decreased reabsorption of fluid and thus HCO3−.
Alterations in Na+ balance, through changes in ECF volume, also have an impact on H+ secretion. With volume contraction (negative Na+ balance), secretion of H+ is enhanced. This occurs via several mechanisms. One mechanism involves the renin-angiotensinaldosterone system, which is activated by volume contraction and leads to enhanced reabsorption of Na+ by the nephron (see Chapter 34). Angiotensin II acts on the proximal tubule to stimulate the apical membrane Na+-H+ antiporter, as well as the basolateral 1Na+-3HCO3− symporter. This stimulatory effect includes increased activity of the transporters and exocytotic insertion of transporters into the membrane. To a lesser degree, angiotensin II stimulates H+ secretion in the early portion of the distal tubule, a process also mediated by the Na+-H+ antiporter. Aldosterone’s primary action on the distal tubule and collecting duct is to stimulate Na+ reabsorption by principal cells (see Chapter 33). However, it also stimulates intercalated cells in these segments to secrete H+. This effect is both indirect and direct. By stimulating Na+ reabsorption by principal cells, aldosterone hyperpolarizes the transepithelial voltage (i.e., the lumen becomes more electrically negative). This change in transepithelial voltage then facilitates the secretion of H+ by the intercalated cells. In addition to this indirect effect, aldosterone acts directly on intercalated cells to stimulate H+ secretion. The precise mechanism or mechanisms for this stimulatory effect are not fully understood.
Another mechanism by which ECF volume contraction enhances H+ secretion (HCO3− reabsorption) is via changes in peritubular capillary Starling forces. As described in Chapters 33 and 34, ECF volume contraction alters the peritubular capillary Starling forces such that overall proximal tubule reabsorption is enhanced. With this enhanced reabsorption, more of the filtered load of HCO3− is reabsorbed.
Parathyroid hormone (PTH) has both inhibitory and stimulatory effect on renal H+ secretion. Acutely, PTH inhibits H+ secretion by the proximal tubule by inhibiting the activity of the Na+-H+ antiporter and by also causing the antiporter to be endocytosed from the apical membrane. Long-term, PTH stimulates renal acid excretion by acting on the thick ascending limb of Henle’s loop and the distal tubule. Because secretion of PTH is increased during acidosis, this long-term stimulatory effect on renal acid excretion is a component of the renal response to acidosis. The stimulatory effect of PTH on acid excretion is due in part to the delivery of increased amounts of Pi to more distal nephron sites, where it is then titrated and excreted as titratable acid.*
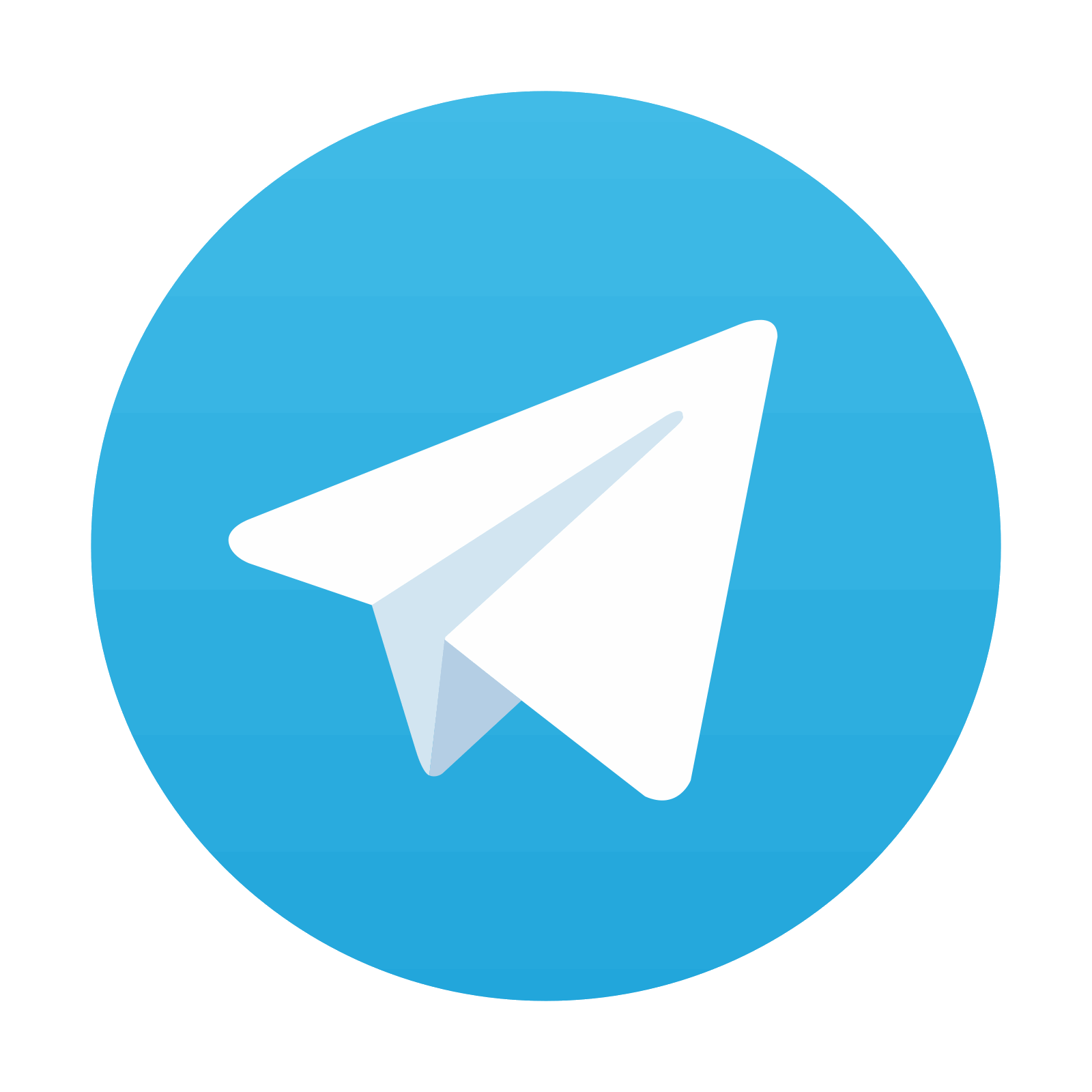
Stay updated, free articles. Join our Telegram channel
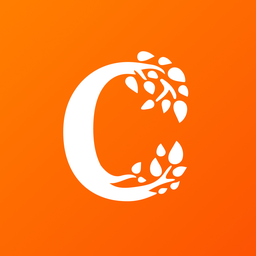
Full access? Get Clinical Tree
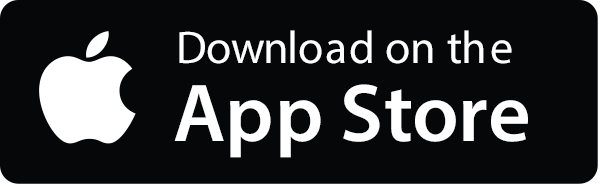
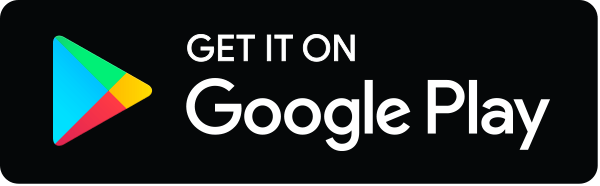