Respiratory Function and Alterations in Gas Exchange
Lorna L. Schumann
Key Questions
• How do the structures involved in gas exchange in the lungs differ from conducting structures?
• What factors determine the work of breathing?
• How are alveolar ventilation and oxygenation estimated and assessed?
• What factors affect the distribution of ventilation and perfusion in the lungs?
• How are oxygen and carbon dioxide transported in the circulation?
• What pathophysiologic factors might alter ventilation-perfusion matching in the lungs?
• What are the risk factors and complications of pulmonary venous thromboembolism and hypertension?
http://evolve.elsevier.com/Copstead/
The primary function of the lungs is gas exchange. Oxygen is transported to the body tissues, and carbon dioxide, a waste product, is transported out of the body. The exchange of these gases takes place at the alveolar-capillary membrane. For effective gas exchange to occur, the processes of ventilation, perfusion, and diffusion must occur simultaneously at the alveolar-capillary interface. Problems with any of these three processes can result in hypoxemia (low arterial oxygen concentration) or hypercarbia (high arterial carbon dioxide concentration). An understanding of the anatomy and physiology of pulmonary gas exchange is necessary for learning about the pathophysiologic processes that follow.
Functional Anatomy
Development of the Pulmonary System
Initially, the laryngotracheal diverticulum includes the esophagus and the trachea as a single tube. Then longitudinal ridges begin to develop along the tube and form a septum (wall), which separates the esophagus from the trachea. Failure of this septum to develop leads to a tracheoesophageal fistula (abnormal opening), leaving a communication between the esophagus and the trachea. This abnormality occurs about once in every 2500 births. Approximately 90% of the cases of esophageal atresia (blind pouch) are of the type seen in Figure 21-1, A. Parts B through D show variations of tracheoesophageal fistulas.1
As the laryngotracheal tube continues to elongate, the lung bud divides into two bronchial buds, which become the bronchi and the right and left lungs. The right bronchus becomes larger than the left. The right mainstem bronchus is normally more vertical than the left because it is the main continuation of the laryngotracheal tube and branches off the trachea at a 20-degree angle. The left bronchus branches off the trachea at an angle of 40 to 60 degrees. This normal anatomic development increases the chances that an inhaled foreign body will lodge in the right mainstem bronchus rather than the left.
Fetal lung development can be divided into the following four periods:
4. The alveolar period (late fetal life to 8 years) is the final period of lung development when alveolar ducts form from terminal sacs and alveoli mature by increasing in size and number. Approximately one eighth to one sixth of the adult number of alveoli are present at birth.1 During this growth period, there is a lack of structural collateral pathways necessary for maintaining open airways. This may make the individual more susceptible to atelectasis (incomplete expansion) and obstruction. Lung damage during this period may cause permanent defects in lung development.1
Upper Airway Structures
The respiratory system can be divided into two major anatomic areas: the upper airway and the lower airway. The upper airway consists of the nasopharyngeal cavity (nasopharynx, oropharynx, laryngopharynx) (Figure 21-2). The lower airway contains the larynx, trachea, bronchi, bronchopulmonary segments, terminal bronchioles, and the acinus (the alveolar region supplied by one terminal bronchiole, which includes numerous alveoli) (see Figure 21-2).
The nasal cavity conducts gases to and from the lungs, and filters, warms, and humidifies the air. It is a rigid box composed of two-thirds cartilage and one-third bone, which prevents collapse during movement of air. The convoluted turbinates (cone-shaped bones) of the nasal cavity are highly vascular, and their blood flow forms an efficient heat exchanger. Evaporation of water from the turbinate surface and from the mucus secreted by mucosal glands raises the water vapor of the inspired air to normal saturation. Therefore, air is warmed to body temperature and humidified.
Air is filtered by the large hairs (vibrissae) of the nasal cavity and cilia that line the nasal cavity. The cilia sweep foreign particles trapped by mucus into the nasopharynx, where they are swallowed or expectorated. An electron micrograph of the tracheobronchial lining is shown in Figure 21-3. Pseudostratified ciliated columnar epithelium lines the trachea and bronchi. Goblet cells and mucus-producing glands are contained in this area and are responsible for synthesizing approximately 100 ml/day in the adult, more with disease. The composition of mucus is 95% water with the remaining 5% consisting of mucopolysaccharides, mucoproteins, and lipids. Maintenance of water content and fluid balance is important to the mobilization of secretions. A child has more mucus-producing glands and therefore produces more mucus than an adult. Consequently, in an ill child the overproduction of mucus in combination with small airway size may precipitate tracheobronchial obstruction.2,3
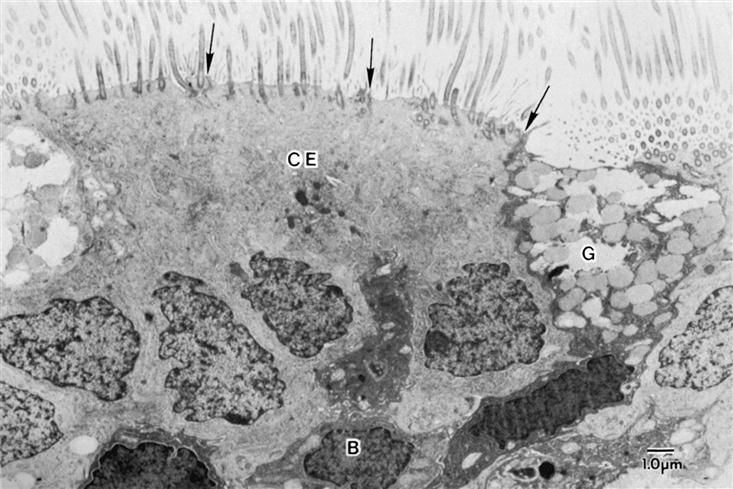
Goblet cells have abundant mucus granules in the cytoplasm, and their apical surface is devoid of cilia. Basal cells, as their name indicates, are located along the abluminal portion of the lining epithelium, adjacent to the basal lamina. The arrows at the apical surface of the airway cells indicate the location of junctional complexes between contiguous epithelial cells. (Human lung surgical specimen, transmission electron microscopy.) (From Murray JF, Nadel JA: Textbook of respiratory medicine, ed 4, Philadelphia, 2005, Elsevier.)
Cilia (Figure 21-4) beat in a sweeping motion like oars rowing a boat at approximately 1000 to 1500 strokes per minute.2,3 Mucociliary transport (movement of mucus upward) is a primary defense mechanism of the tracheobronchial tree. Inhaled particles, bacteria, and macrophages are removed from the respiratory tract by ciliary clearance and the cough reflex. Ciliary function is impaired by smoking, alcohol ingestion, hypothermia, hyperthermia, cold air, low humidity, starvation, anesthetics, corticosteroids, noxious gases, the common cold, and increased mucus production.2,3
The four paranasal sinuses are air-containing spaces adjacent to the nasal passages that provide speech resonance and increase the surface area for heat and water vapor exchange. The sinuses are swept clean by mucociliary action when the communicating passages that connect them with the nasal passages remain open.
The eustachian tube between the middle ear and the posterior nasopharynx maintains the air in the middle ear at atmospheric pressure. To prevent secretions or food from entering the middle ear during swallowing, the pharyngeal muscles close the eustachian tube briefly. The nasal end of the eustachian tube is surrounded by flexible cartilage arranged in a spiral configuration. The muscles surrounding the eustachian cartilage close the opening by pulling the cartilage tighter. Because the tube is shorter in children, the potential for otitis media (infection of the middle ear) is increased.2,3
Lower Airway Structures
After air passes through the nasal cavity or oral cavity into the pharynx, it moves into the larynx and finally into the tracheobronchial tree. The acinus (Figure 21-5) is located at the end of the tracheobronchial tree and is composed of bronchioles, alveolar ducts, and alveoli.
The larynx is the transition area between the upper and lower airways. Anatomically it is considered part of the lower airway, but functionally it is similar to the upper airway. The larynx contains the epiglottis, vocal cords, and cartilages. The anatomic arrangement of the larynx functions to prevent aspiration during swallowing and to assist in phonation and coughing. Each vocal cord is attached anteriorly to the thyroid cartilage and posteriorly to the arytenoid cartilage. Vibration of the cords leads to phonation. Food is prevented from entering the trachea during swallowing by closure of the epiglottis. If food or fluid should bypass the epiglottis and enter the tracheobronchial tree, the cough reflex is initiated. The majority of cough receptors lie at the carina. A cough reflex is produced when the epiglottis and vocal cords close tightly against air entrapped in the lungs. Occasionally, individuals cough hard enough to break a rib. When the expiratory muscles contract forcefully against the closed epiglottis and vocal cords, a pressure of approximately 100 mm Hg is created. When the cords and epiglottis suddenly open, the high-pressure buildup is allowed to escape. This reflex rapidly removes foreign matter from the tracheobronchial tree.2,3
The major cartilages of the larynx are the thyroid, cricoid, and arytenoid. The thyroid cartilage is a large shield-shaped cartilage often referred to as the Adam’s apple. Immediately below the thyroid cartilage is the site for emergency opening (cricothyroidotomy) of the tracheal passageway. The cricoid cartilage lies below the thyroid cartilage and is the narrowest point in the airway of a child. It is the only complete tracheal ring, and because of its narrowness in the small child’s airway, an endotracheal tube cuff is not necessary for required intubation of the airway.
The trachea, bronchi, and bronchioles make up the conducting airways that allow passage of gases to and from the gas exchange units (alveoli). These conducting airways comprise a proportionately larger amount of the total airway system in the infant and child than in the adult. The trachea (Figure 21-6) contains incomplete cartilaginous rings; it is approximately 11 to 13 cm long and lies between the cricoid cartilage and the carina (ridge located at the lower end of the trachea). Individual variations in tracheal shape include U, circular, D, C, triangular, and elliptical (Figure 21-7). Of 111 adult tracheas studied, the incidence of shapes in order of frequency was 48.6% C, 27% U, 12.6% D, 8.2% elliptical, 1.8% circular, and 1.8% triangular.4 These tracheal variations may affect ventilation of patients who have endotracheal tubes in their airways and require mechanical ventilation.
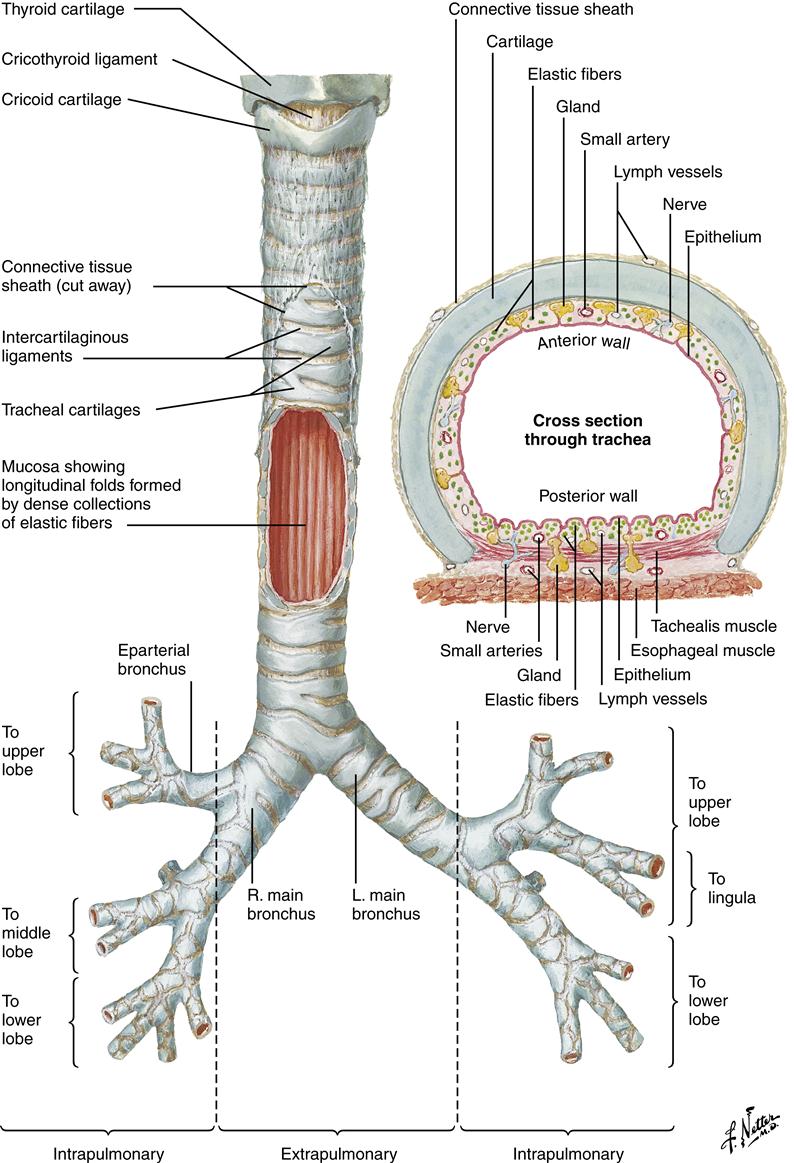
L, Left; R, right. (Netter illustration from www.netterimages.com. Copyright © Elsevier Inc. All rights reserved.)
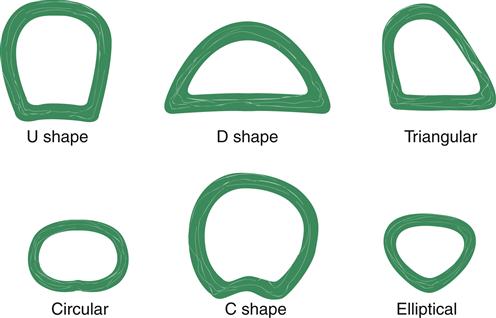
The trachea divides into two mainstem (primary) bronchi, which contain cartilage and smooth muscle. Viewing the body anteriorly, the carina is located at the angle of Louis, between the sternum and manubrium at the second intercostal space.
The small size of the conducting airway in the infant and child makes even a small decrease in the size of the lumen from an obstruction critical to airway conduction.5 Primary bronchi further divide into five (secondary) lobar branches, three to the right lung and two to the left lung. Each lobar branch enters a lobe of the lung and further divides into bronchopulmonary segments (10 segments in the right lung, 9 segments in the left lung) (Figure 21-8).2,3 Each bronchopulmonary segment is composed of 50 or more terminal bronchioles (conducting airways), which branch into respiratory bronchioles, where gas exchange begins.
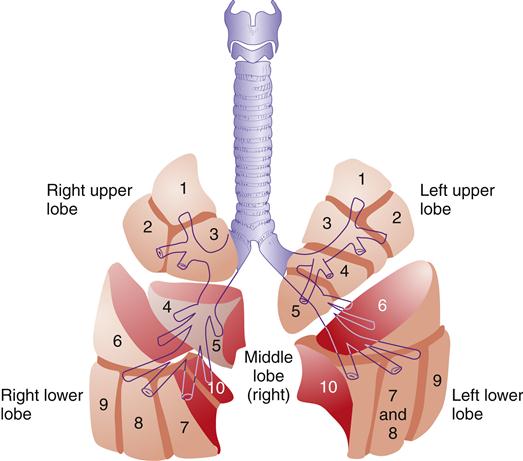
Right and left upper lobes: 1, apical segment; 2, posterior segment; 3, anterior segment. Left upper lobe: 4, superior segment; 5, inferior segment. Middle lobe (right): 4, lateral segment; 5, medial segment. Right and left lower lobes: 6, superior (apical) segment; 7, medial basal segment; 8, anterior basal segment (on left, 7 and 8 combine to form the anteromedial basal segment); 9, lateral basal segment. (10, posterior basal segment visible in medial view; not shown here.)
Terminal bronchioles, which include the conducting airways, further subdivide into two or more respiratory bronchioles in which gas exchange begins. The respiratory bronchioles divide into two or more alveolar ducts, which in turn supply several alveoli.
Nervous system control of the bronchi and bronchioles is mediated by the autonomic nervous system. Stimulation of the parasympathetic nervous system via the vagus nerve leads to constriction (by means of acetylcholine receptors) of bronchial smooth muscle. Stimulation of the sympathetic nervous system leads to relaxation of bronchial smooth muscle. Sympathetic stimulation is mediated by β2-adrenergic receptors, which are under the control of circulating catecholamines.5 (See the discussion in the Neurologic Control of Ventilation section later in this chapter for additional information.)
The lung is fully developed by the eighth year of life.1,2 The large alveolar surface area in conjunction with pulmonary surfactant, a phospholipid produced by type II alveolar cells, lowers surface tension and facilitates gas exchange. Two other types of cells are found: type I alveolar cells (type I pneumocytes), which are the epithelial structural cells of the alveoli, and alveolar macrophages, which act as a defense mechanism by phagocytizing particles in the alveoli. Alveolar macrophages can be damaged by cigarette smoking and by inhalation of silica (SiO2).
Adult lungs contain approximately 300 million alveoli, and the newborn lung contains one eighth to one sixth the adult number.1–3 An elderly person may also have a reduction in the number of alveoli as part of the normal aging process, but many elderly people retain the same number of alveoli they had as a younger adult.
Gas exchange occurs in the alveolar units (see Figure 21-5) where oxygen (O2) and carbon dioxide (CO2) transfer across the alveolar-capillary membrane. The partial pressures of gases in the alveoli are termed PAO2 for oxygen and Paco2 for carbon dioxide. The partial pressures of gases in the blood are termed Pao2 for oxygen and Paco2 for carbon dioxide. Collateral alveolar ventilation can also occur through holes in the alveolar walls, called the pores of Kohn or canals of Lambert. A small child has less collateral ventilation, because of fewer pores of Kohn.6 The alveolar membrane is thicker in the neonate and reaches the adult thinness of 0.5 mm by the age of 8 years. This thinner membrane may allow increased transfer of O2. The healthy older adult has very thin-walled, enlarged air sacs and fewer capillaries than a younger adult.2,7 Respiratory system changes associated with normal aging are described in Geriatric Considerations: Changes in the Respiratory System.
Pulmonary Circulation
Blood supply to the lungs comes from two sources: the bronchial artery system, which supplies a small amount of oxygenated blood to the pleura and lung tissues, and the pulmonary artery system, which provides a vast capillary network for O2 and CO2 exchange. The capillary networks of the neonate, young child, and elderly person are less than those in the average healthy adult. Oxygen-depleted (unoxygenated) blood leaves the right ventricle by way of the pulmonary artery trunk, which branches into the right and left pulmonary arteries. The pulmonary arteries further divide into smaller arteries and arterioles that feed into the capillary network where gas exchange occurs from the alveolar-capillary membrane. Pulmonary artery blood is unoxygenated, and blood in the pulmonary veins is oxygenated. The opposite is true in the rest of the body, where the arterial blood is oxygenated and the venous blood is unoxygenated.
The capillary network is a low-pressure system that can expand two to three times the normal size before a significant increase in pulmonary capillary pressures is detectable. The normal pulmonary arterial pressure in a healthy adult is about 22/8 to 25/8 mm Hg. The mean pulmonary arterial pressure is approximately 15 mm Hg. This compares with the high pressure of the systemic circulation, which is normally considered to be 120/80 mm Hg, with a mean arterial pressure of 96 mm Hg.
Under normal resting conditions, some pulmonary capillaries are closed and not perfused (filled with blood). The pulmonary circulation has two mechanisms for lowering pulmonary vascular resistance, when vascular pressures are increased because of increased blood flow (Figure 21-9).2,3,5 The first mechanism is recruitment, which allows opening of previously closed capillary vessels. The second mechanism is distention, which allows for widening of capillary vessels.
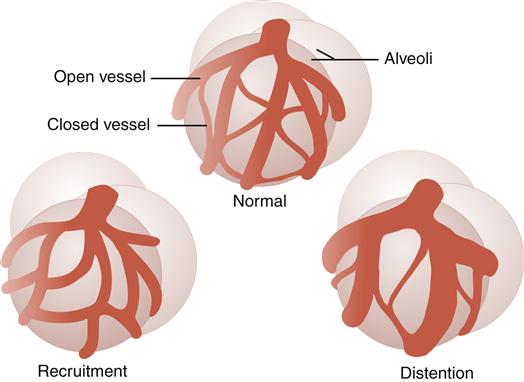
Recruitment allows for opening of previously closed capillaries. Distention allows for widening of capillary vessels.
Another factor influencing pulmonary circulation is the fluid balance of the lung tissues. Fluid balance is regulated by the hydrostatic pressure, colloid osmotic pressure, and capillary permeability. When capillary hydrostatic pressure exceeds colloid osmotic pressure, fluid moves from the capillary to the interstitium. If the fluid shift is not controlled, the fluid volume will continue to increase until fluid is moved into the alveoli. Alveolar edema is more serious than interstitial edema (fluid in the interstitial space), because of its negative effects on gas exchange. Pulmonary interstitial and alveolar edema is common in disease processes such as congestive heart failure and infectious diseases of the lung. Other disease processes that also increase capillary permeability are acute respiratory distress syndrome (ARDS) and infant respiratory distress syndrome. (See Chapter 23 for further discussion.)
Age-Related Variations
Structural and physiologic variations occur at each end of the age continuum. A summary of anatomic and physiologic respiratory variations by age group is presented in Table 21-1.8Pediatric considerations are shown in the box below.
TABLE 21-1
VARIATIONS IN ANATOMY AND PHYSIOLOGY OF THE RESPIRATORY SYSTEM BY AGE GROUP
YOUNG NEWBORNS | CHILDREN | ADULTS | ELDERLY (OVER 60 YEARS OLD) | |
Pao2 (mm Hg) | 60-70 | 90-100 | 90-100 | 70-80 |
Paco2 (mm Hg) | 45-50 | 35-45 | 35-45 | 35-45 |
pH | 7.3-7.4 (depends on Apgar score) | 7.35-7.45 | 7.35-7.45 | 7.30-7.45 |
Bicarbonate (mEq/L) | 20-26 | 22-28 | 24-30 | 24-30 |
Anatomic dead space | Proportional to size | Proportional to size | ≈150 ml | ≈150-200 ml |
Number of alveoli | 12.5-16.5% of adult number | Adult number by 8 years old | 300,000/lung | ≤300,000/lung |
Thickness of alveolar membrane | Thicker than adult | Adult by 8 years old | <0.5 μm | Thinner than adult |
Number of capillaries | Less than adult | Adult by 8 years old | Adult | Less than adult |
Vital capacity | Proportionately less than adult | Proportional to size | 4.7 L | Less than adult |
Tidal volume | Proportional to size | Proportional to size | 500 ml | Less than adult (30% less by age 80 years) |
Compliance | More compliant than adult | Similar to adult | Static compliance (90-100 ml/cm H2O) | Less compliant |
Airway resistance | Greater than adult | Greater than adult | 1.0-1.5 cm H2O/L/sec | Adult level or less |
Data from Fretwell ME: Aging changes in structure and function. In Carnevali DL, Patrick M, editors: Nursing management for the elderly, ed 3, Philadelphia, 1993, Lippincott.
Ventilation
Lung Volumes and Capacities
Ventilation is the process of moving air into the lungs and distributing air within the lungs to gas exchange units (alveoli) for maintenance of oxygenation and removal of carbon dioxide (CO2).5 Measures of ventilation (amount of air moved) include four lung volumes and four lung capacities. Figure 21-10 schematically presents the various lung volumes and capacities; Table 21-2 defines each term and provides further details.
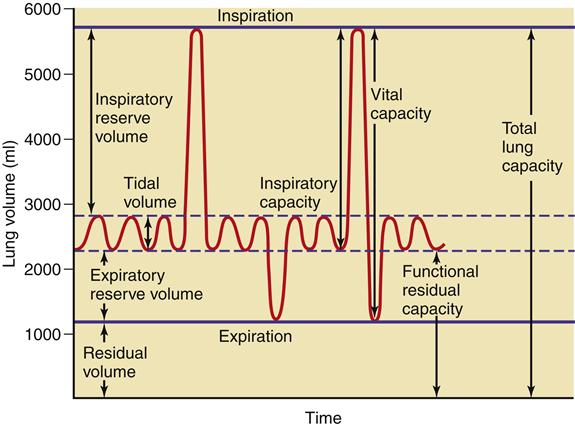
TABLE 21-2
TERM | DEFINITION (TYPICAL VOLUME) |
Lung Volumes | |
Tidal volume | A normal breath (≈500 ml) or amount of gas entering or leaving lung during normal breathing |
Inspiratory reserve volume | Amount of gas a person is able to inspire above a normal breath (e.g., maximal deep breath, ≈3 L) |
Expiratory reserve volume | Amount of gas expired beyond tidal volume (≈1.2 L) |
Residual volume | Volume of gas left in lungs at end of a maximal expiration (≈1.2 L) |
Lung Capacities | |
Vital capacity | Total volume of gas that can be exhaled during maximal expiration (≈4.8 L) |
Inspiratory capacity | Amount of gas that can be inspired from a resting expiration (≈3.5 L) |
Functional residual capacity | Amount of gas left in lungs at end of a normal expiration (≈2.4 L) |
Total lung capacity | Amount of gas contained in lungs at maximal inspiration (≈6.0 L) |
Lung volumes and capacities vary according to the individual’s body size, age (decreased in the neonate, young child, and the elderly),7–9 and body position (supine versus upright). Testing of pulmonary function to measure these volumes and capacities is covered under the Diagnostic Tests section in Chapter 22. Other measures important to ventilation are dead space, minute ventilation, and alveolar ventilation.
Dead Space
Dead space includes three dimensions: anatomic dead space, alveolar dead space, and physiologic dead space. Anatomic dead space includes the volume of gas (not used in gas exchange) in the conducting airways from the nose to the respiratory bronchioles.5,10,11 Generally, in adults this area is equal to 1 ml per pound of ideal body weight, or approximately 150 ml. In newborns and young children, the anatomic dead space is proportionately larger for their size.6 The anatomic dead space of elderly persons may increase slightly over that of healthy young adults because of the loss of alveolar sacs. Alveolar dead space is composed of ventilated, but unperfused areas of the lung, and is often referred to as wasted ventilation.5 Physiologic dead space (functional dead space) is the sum of the anatomic dead space and alveolar dead space.7,11 Approximately one third of each breath occupies dead space.
Minute Ventilation
Minute ventilation is the product of tidal volume (milliliters of air inhaled with each breath) times respiratory rate per minute. For example, a person with a tidal volume of 500 ml who is breathing at a rate of 15 breaths/minute has a minute ventilation of 7500 ml (see Table 21-2 for typical volumes).
Alveolar Ventilation/Oxygenation
By comparison, alveolar ventilation () equals the difference between tidal volume (VT) and anatomic dead space volume (VD) multiplied by the respiratory rate (RR) per minute.10

Because alveolar ventilation is affected by both the anatomic dead space and the respiratory rate, slow deep breathing yields greater alveolar ventilation than rapid shallow respiration. The patient breathing 25 times/minute at a VT of 200 ml would have alveolar ventilation as follows:

A patient breathing 10 times/minute at a VT of 600 ml would have an alveolar ventilation of

The partial pressure of oxygen in the alveoli (Pao2) is the driving force to move O2 into the blood and is estimated with the following equation:

where FIO2 is the fraction of inspired oxygen, PB is the barometric pressure, 47 is the constant for water vapor pressure (mm Hg), 0.8 is the respiratory quotient, and Paco2 is the laboratory measurement of arterial CO2 pressure (mm Hg).
The value for PAO2 is normally very close to that for PaO2. The difference between alveolar and arterial oxygen tensions is called the Alveolar-arterial Difference in oxygen (A − aDO2). A large A − aDO2 value indicates poor matching of alveolar ventilation with alveolar blood flow ( matching)
For example, the calculation of A − aDO2 for a person at sea level (PB = 760 mm Hg) breathing room air (FIO2 = 0.21) with Pao2 = 75 mm Hg and Paco2 = 40 mm Hg is

Therefore, using the arterial blood gas value obtained for the PaO2 and the calculated PAO2 of 100, a difference of 25 mm Hg is determined:

This large of a difference indicates a significant problem with gas exchange.
In critical care settings, it is useful to calculate the A − aDO2 value to monitor the efficacy of oxygen exchange across the lung. The normal A − aDO2 gradient in a normal, healthy young adult is less than 10 mm Hg at room air, but it increases with age and increasing FIO2. A rising A − aDO2 value indicates worsening lung function, even though hypoxemia (PaO2 lower than 80 mm Hg at sea level) may not necessarily be present.
Hypoxemia that is primarily caused by hypoventilation suggests that the lung is normal, and treatment that increases ventilation will remedy the problem. This type of hypoxemia is characterized by a normal A − aDO2 value.
A simple method of calculating expected PAO2 is the “law of 5’s.” By multiplying the FIO2 (%) by 5, the care provider has an estimate of what the oxygen level should be under normal, healthy conditions (e.g., 5 × 21% room air = 105).
Mechanics of Breathing
The mechanics of breathing include the concepts of airway resistance, lung compliance, and opposing lung forces (elastic recoil versus chest wall expansion) of the lung. These factors affect the overall performance of gas exchange and the work of breathing.
The lungs have a natural recoil tendency, whereas the chest wall favors the expanded state. During inspiration, the chest wall muscles (external intercostals) contract, elevating the ribs as the diaphragm moves downward. These two actions create a negative intrapleural pressure that causes the lung to expand. During expiration, the lung deflates passively because of the elastic recoil (elastic fibers in the lung tissue) and relaxation of the diaphragm. During heavy breathing, as seen with exercise, the elastic forces are not strong enough to cause the necessary rapid expiration, so abdominal muscles contract, pushing the abdominal contents upward, compressing the lungs.3 Figure 21-11 shows the interaction of lung forces during inspiration and expiration. In the normal, healthy resting individual, expiration is accomplished almost entirely by relaxation of the diaphragm.5 At the end of a normal expiration, the alveoli still contain some air volume, known as the functional residual capacity. If the alveoli were allowed to empty completely, the high surface tension in the alveoli would make it more difficult to reinflate them and add significantly to the work of breathing. In the absence of surfactant, which reduces alveolar surface tension, the alveoli tend to collapse—a condition called atelectasis. Excessive surface tension can increase the work of breathing so much that mechanical ventilation may be required. This is often the case in ARDS and in infant respiratory distress syndrome (see Chapter 23).
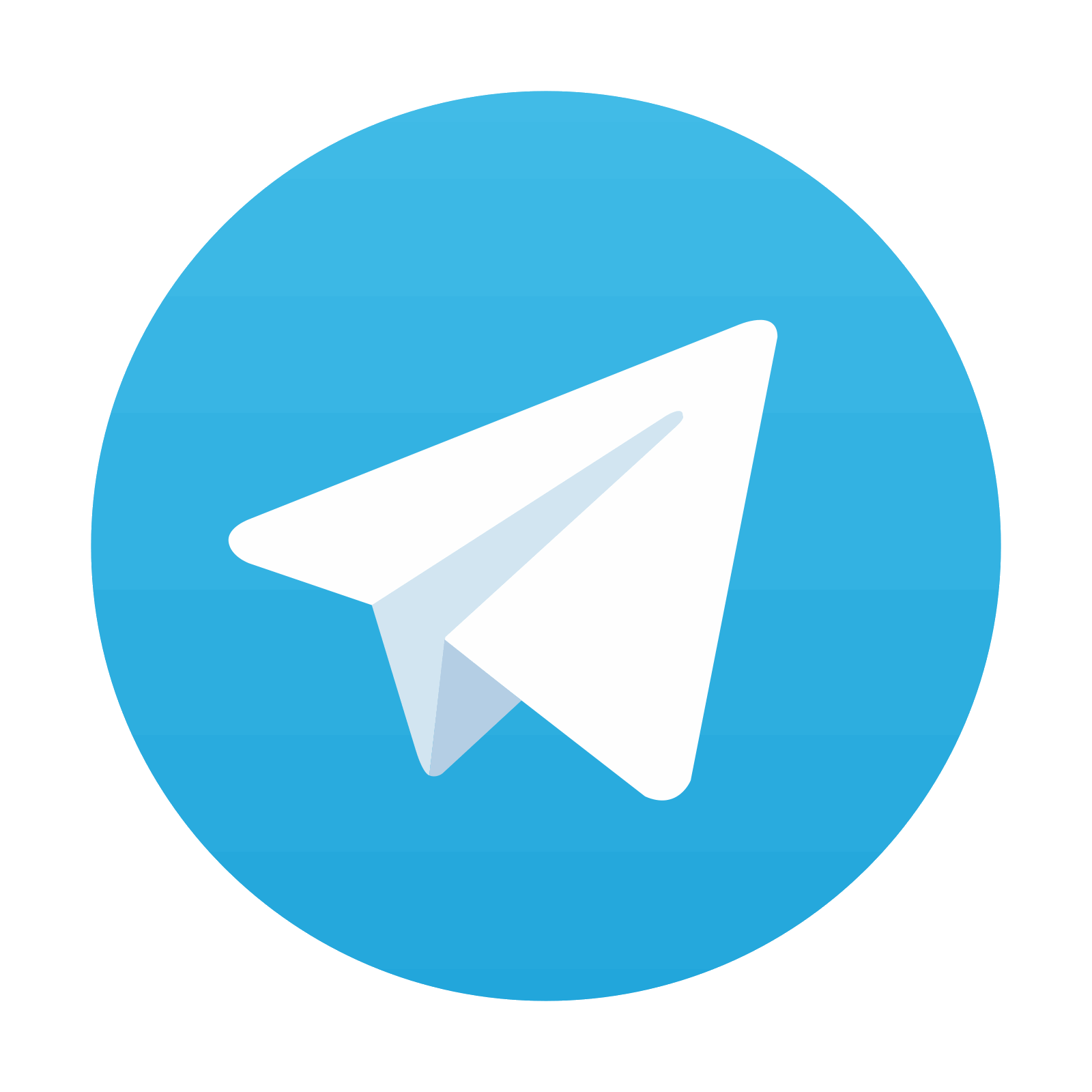
Stay updated, free articles. Join our Telegram channel
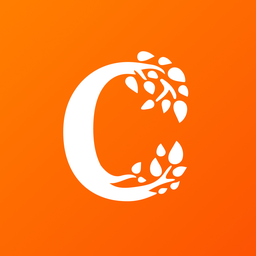
Full access? Get Clinical Tree
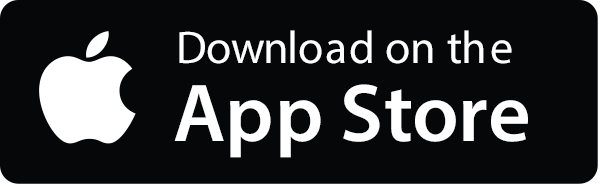
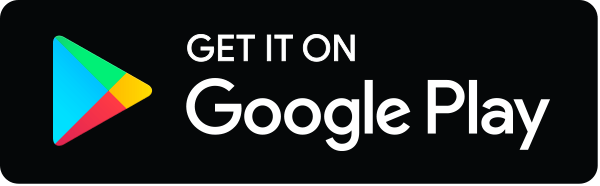