) and prevents the flow of ions between the pipette and the membrane. Transmembrane ion-flux through channels is measured as an electrical current using an electronic amplifier connected to the pipette. The membrane patch within the pipette is disrupted by application of strong suction giving access to the interior of the cell achieving a whole cell configuration that allows measurements of currents from the entire cell membrane. This also allows diffusional exchange between the pipette and the cytoplasm and gives a convenient way to control the intracellular environment and interrogating the regulation of ion channels by intracellular signal transduction mechanisms.
Two electrode voltage clamp technique is another popular voltage clamp method, especially to study ion channels expressed in Xenopus oocytes using recombinant techniques.6 Two sharp microelectrodes impale the cell and achieve access to the intracellular environment. One electrode measures the membrane potential and electronically compares this to the command potential set by the amplifier. The electronic feedback circuit then passes a current into the cell through the second electrode to maintain the clamp and simultaneously measures the current.
In current clamp experiments, the amplifier records the spontaneous and evoked changes in the membrane potential of the cell. The spontaneous changes are generated in the cell in the absence of stimulation. Evoked potentials arise as a result of electrical, mechanical or chemical stimulation of the cell. This technique is useful to study the electrical properties of cells and tissues in their native environment. Some examples include recording myocardial or neuronal action potentials in in vitro and ex vivo preparations where the tissue architecture is preserved. The membrane potential is recorded using intracellular or extracellular electrode placements.
Single-unit recording involves the placement of a microelectrode in the proximity of an excitable cell (e.g. neurons) either in vitro, ex vivo or in vivo to detect the activity of a single cell. The action potentials recorded as single-unit recordings are similar to the intracellularly recorded action potentials but are much less in amplitude. Single-unit recordings from neurons in living animals or in ex vivo preparations provide valuable insights into physiological and neurochemical processes.
The next two sections provide an introduction to both these experimental techniques. A systematic approach to ion channel investigations using electrophysiological techniques is briefly described. Section 2 describes voltage clamp technique to measure ionic currents and study biophysical properties of channels and pharmacology of drugs. Section 3 focuses on measurement of action potentials and describes single fibre recordings.
Consistent with the theme of this book, these sections illustrate a hypothesis-driven approach to ion channel investigation. They involve researching the background, framing the objectives, formulating an experimental hypothesis, asking tangible questions, conducting the experiments, analysing the data, interpreting the results and discussing the outcomes of the study.
Section 2: Voltage Clamp
This section outlines the protocols used in electrophysiology for assessment of biophysical properties of voltage-gated channels and pharmacology of drugs and the infrastructure of the laboratory needed to conduct patch clamp experiments.

Protocol 1
Title: Modulation of Kv1.x potassium channels by auxiliary β-subunits
Background
Potassium channels constitute a superfamily of transmembrane proteins that form potassium selective pores and play an important role in many physiological functions. Voltage-gated potassium channels (Kv1) are tetrameric proteins that are gated by changes in transmembrane potential and play an important role in the repolarisation of excitable cells. The pore of Kv1 channels consists of four α-subunits assembled as either homo- or heterotetramers. The activity of the α-subunit is modulated by auxiliary intracellular β-subunits.7 The modulatory effects are on the current amplitude as well as on the kinetics. Three different Kvβ subunit genes (β1, β2 and β3) have been cloned from different tissues in several species. Kvβ subunits coassemble with Kvα subunits in the endoplasmic reticulum and enhance biosynthesis, maturation and surface expression of voltage-gated Kv1 channel complexes.7 Kvβ2 increases expression of Kv1.28 and Kv1.49 in the plasma membrane and enhances currents. The N terminus of Kvβ1.x (β1.1, β1.2 and β1.3) acts as a ball peptide and rapidly inactivates open channels. This fast inactivation resembles the N-type inactivation produced by the N-terminal ball peptide of Kvα subunits. The Kvβ2 subunit while lacking the inactivation peptide accelerates the N-type inactivation of Kv1.4 and, in addition, increases Kv1.4 expression.
Objectives
Primary
Secondary
To investigate the coexpression of Kv1.x with Kvβ1.2 and/or Kvβ2 in rat brain and heart.
Hypotheses
Research Questions
Research Methodology
Isolation of Xenopus Oocytes and Injection of cRNA
Xenopus oocytes are stored in the ovarian lobes in the abdominal cavity in clumps made up of oocytes, connective tissue, blood vessels and follicle cells. The ovarian lobes are surgically removed from the abdominal cavity and the oocytes are isolated from the lobes by collagenase treatment followed by manual removal of the follicular layer under a microscope using fine forceps. Stage V and VI oocytes that are large in size are routinely used in electrophysiological experiments.6 The mRNA is injected only in healthy defolliculated oocytes. The concentration of Kv1.x cRNA to be used in experiments is determined by titrating it in a pilot experiment while simultaneously monitoring the expression of Kv1.x currents. The final concentration of Kv1.x cRNA should display an optimal channel expression and current amplitudes that are accurately measured by the electronics without introducing clamp artefacts. The concentrations of Kvβ cRNA also need to be titrated using a fixed concentration of Kv1.x cRNA. On the basis of published literature, a starting concentration of 50 ng/µL is most commonly used.
Two-Electrode Voltage Clamp Studies
Whole cell currents are measured at room temperature (20°C–23°C) using the standard two-electrode voltage clamp technique. Electrodes are filled with 3 mol/L KCl and have a resistance of 0.2–0.5 m when immersed in a bath solution. A typical bath solution composition is as follows (in mmol/L): 50 KOH, 55 NaOH, 0.5 CaCl2.2H2O, 100 methanesulfonic acid, 2MgCl2.6H2O and 10 HEPES, pH 7.3. A time course of current expression is monitored after cRNA injection and measurements are done in an optimal window to maintain consistency. Typical voltage protocols used to assess Kv1 family of potassium channels are illustrated in Figure 6.1.
Data Analysis
Current–voltage relationship is obtained by plotting the peak current amplitude in each voltage step as a function of voltage (Figure 6.1A). Current kinetics should monitor for changes in the rate of activation, decay of steady state current and the deactivation kinetics when the voltage returns to resting state. Steady state inactivation is measured by analysing the current amplitude in P2 as a function of the voltage applied in P1 (Figure 6.1B) and fitting it into the Boltzman equation I/Imax = 1/[1 + Exp(Vm − V0.5)/k], where ‘Vm’ is the test potential, ‘V0.5’ is the potential for half activation and ‘k’ is the slope factor. Recovery from inactivation is measured by plotting ratio of currents P2/P1 (Figure 6.1C) as a function of the time lapsed (∆T) between the two pulses. Recovery from inactivation is fit into a single or double exponential fit equation.
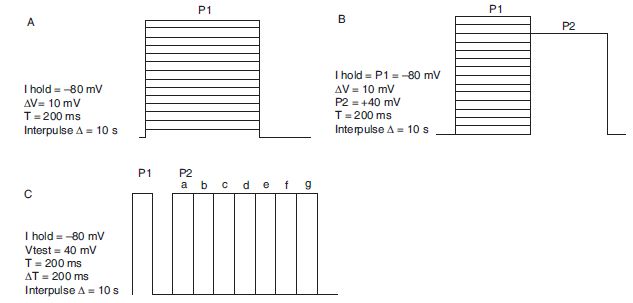
Figure 6.1. Voltage protocols for studying biophysical properties of Kv1 potassium channels. Holding potential, test pulse potential, duration and frequency of stimulation are illustrated next to the waveforms. (A) Current–voltage relationship. (B) Voltage-dependence of inactivation—a series of prepulses (P1) from −80 mV to +60 mV in 10 mV increments are applied prior to a test pulse (P2) to +40 mV. The current evoked by the P2 provides an assay for the extent of inactivation during the prepulse. (C) Recovery of channels from inactivation is measured using paired pulses separated by different time intervals.
Protocol 2
Title: Methods in safety pharmacology: Investigating drug interactions with hERG potassium channels by patch clamp technique
Background
Potassium channels play an important role in cardiac action potential repolarisation. The IKr (hERG) and IKs (KVLQT1/mink) currents in particular are essential for the Phase III of repolarisation and termination of action potential. Congenital mutations in genes encoding hERG or KVLQT1 channels or their accessory subunits have been shown to cause repolarisation defects; action potential prolongation accompanied with the prolongation of QT interval in an ECG10–12 and can predispose individuals to potentially fatal polymorphic ventricular arrhythmia, Torsades-de-pointes (TDP). Acquired long QT syndrome and TDP can also be induced by non-specific block of hERG channels by compounds of diverse chemical structure, including diverse groups of non-antiarrhythmic drugs (NARDS).13 Drug discovery efforts in the past decade have been plagued by drug-induced inhibition of hERG potassium channel. This has led to withdrawal from market of several NARDS such as astemizole, sertindole, cisapride, terfenadine and grepafloxacin and has made the assessment of cardiac ion channel activity of new chemical entities an integral component of drug discovery programmes. In vitro assessment of the effects of new pharmaceutical entities on hERG potassium channels14 and in vivo assessment for QT prolongation in telemetered dogs or monkeys is now an integral part of drug discovery.15
Objectives
To test whether a new antidiabetic drug A has torsadogenic liability.
Hypothesis
All NARDS that produce TDP inhibit hERG potassium channels, prolong cardiac action potentials and prolong QT interval of the ECG. Compound A does not inhibit hERG currents and is unlikely to produce TDP.
Research Questions
Research Methodology
Cell Culture
Any cell line that stably expresses the hERG potassium channel is used as a test system. Cells are maintained in a monolayer culture in defined media at 37°C in a humidified incubator with an atmosphere containing 5% CO2. Cells for electrophysiological studies are seeded on glass coverslips.
Solutions and Chemicals
Normal extracellular Tyrode solution contains (mmol/L) NaCl (140), KCl (4), MgCl2.6H2O (1), CaCl2.2H2O (2), HEPES (10); pH 7.4, osmolarity 296–300 mOsm. Internal recording solution contains (mmol/L): K+ aspartate (130), KCl (10), CaCl2.2H2O (0.1), MgCl2.6H2O (1.0), HEPES (10), K4BAPTA (5), MgATP (5); pH 7.2, osmolarity 280–285 mOsm. Compound A dilutions are made in DMSO and the final concentration of DMSO in test solution is ≤0.1%.
Voltage Protocols
As hERG currents exhibit rapid inactivation, most protocols to assess hERG pharmacology employ measurement of tail currents at potentials where there is no voltage-dependent inactivation and the channels are slowly returning from a fully activated state to a deactivated state. Voltage protocols are obtained from the literature. Prototypic examples are shown in Figure 6.2.
Data Analysis
Peak tail currents in the absence and presence of compound A are measured. Percentage inhibition is plotted as a function of the compound concentration. Data are plotted as mean ± standard error of mean (SE). Concentration–response curves are fit to the Hill equation: IDrug/IControl = 1/[1 + (X/IC50)n], where ‘I’ indicates current, ‘X’ indicates the concentration of drug, IC50 is the concentration of drug required for 50% inhibition of current and ‘n’ is the Hill coefficient.
Activation curves are fit to the Boltzman equation I/Imax = 1/[1 + Exp(Vm − V0.5)/k], where ‘Vm’ is the test potential, ‘V0.5’ is the potential for half activation and ‘k’ is the slope factor.
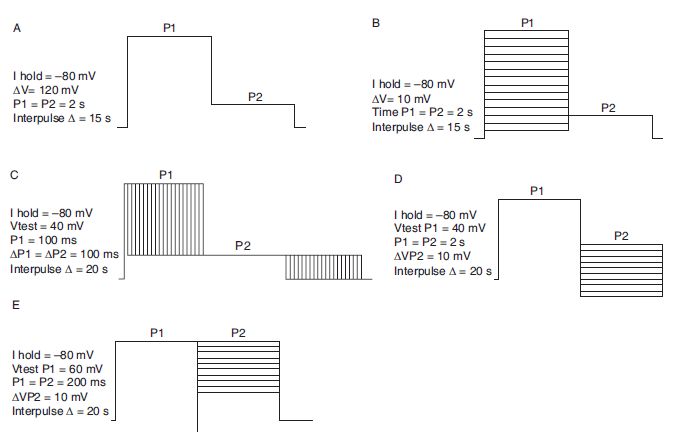
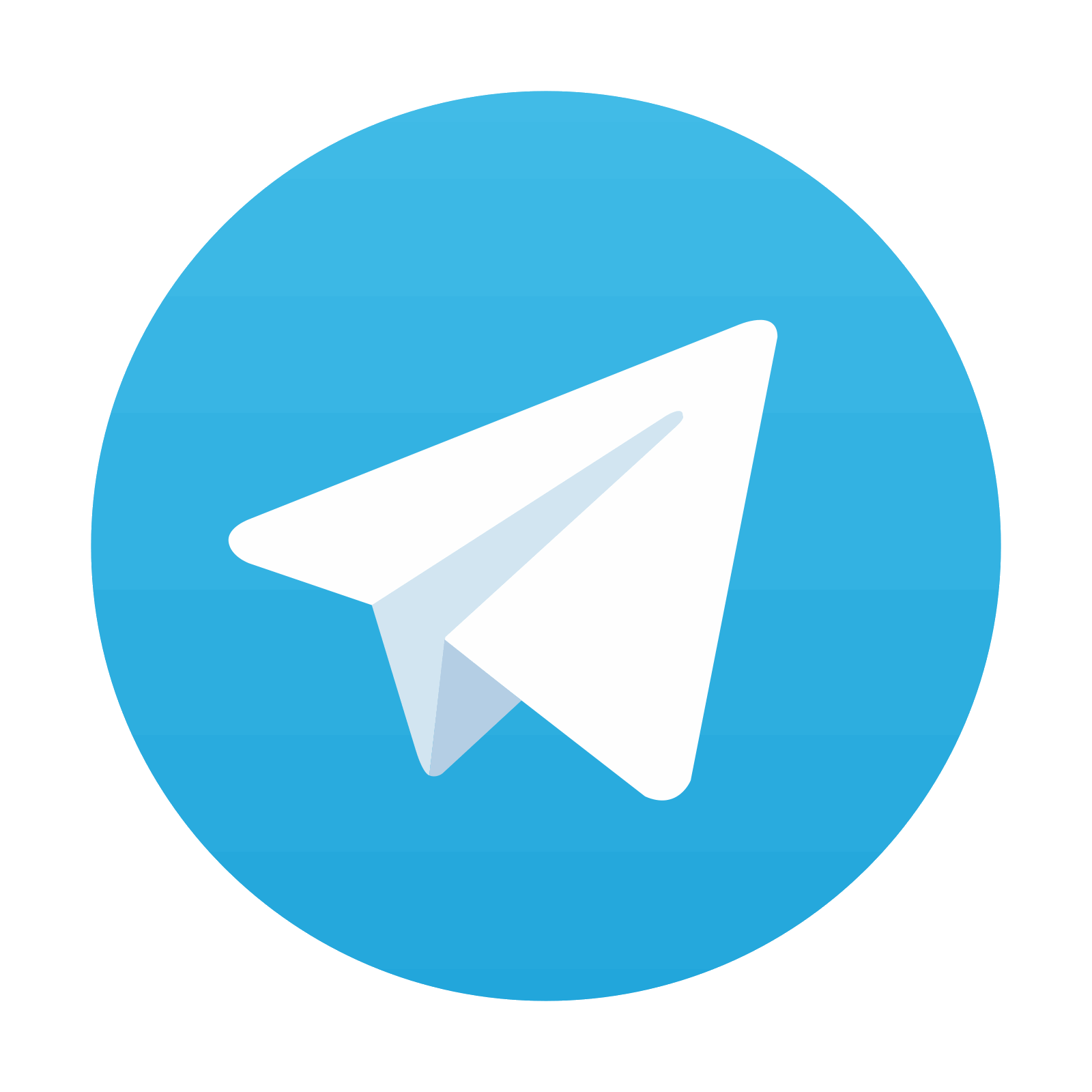
Stay updated, free articles. Join our Telegram channel
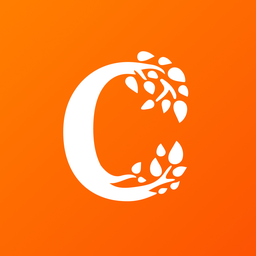
Full access? Get Clinical Tree
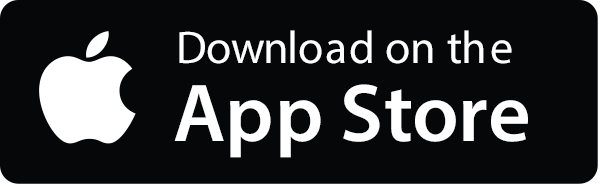
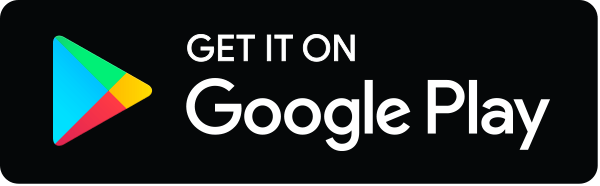