Chapter 19
Regulation of Fuel Utilization in Response to Food Intake
In addition to the overall fuel needs of the body, the synthesis, oxidation, and storage of each of the individual macronutrients must be regulated to meet the specific needs of the various body tissues. In the context of fuel metabolism, different tissues have unique functions and metabolic pathways and also are exposed to circulating fuels in differing ways. For example, the portal blood draining the gastrointestinal tract is the major blood supply to the liver such that the glucose and amino acid supply to the liver during the absorptive period exceeds the fuel needs of the liver itself, and the liver plays a key role in modulating the mixture of circulating fuels that are supplied to the rest of the body. In addition, some tissues require specific types of fuel molecules. Red blood cells, which do not contain mitochondria, cannot obtain energy by oxidizing fatty acids, but they can generate ATP by converting glucose to lactate, which is released back into the circulation. If plasma glucose levels are not maintained, tissues dependent upon glucose as a fuel will suffer damage. Without energy, cells no longer function and eventually die, resulting in tissue injury. Lack of fuel can even result in permanent brain damage, stroke, and death. The brain is capable of using only glucose and ketone bodies as major fuels; although brain cells have mitochondria, the blood–brain barrier in key regions of the brain limits the rate of transfer of long-chain fatty acids. Thus, long-chain fatty acids are not a major fuel for the brain even when their level in the circulation is elevated (Vannucci and Hawkins, 1983). Ketone body production by the liver plays a key role in reducing brain glucose use during prolonged starvation. Thus the body must maintain a mixture of circulating fuel molecules that will meet the needs of all tissues, and this might be remarkably different from the mixture absorbed from the gastrointestinal tract following a meal or the mixture released from endogenous stores of fat and glycogen during starvation. Table 19-1 summarizes the main circulating fuels that are used and released by various tissues.
TABLE 19-1
TISSUE | FUELS USED | FUELS RELEASED |
Brain | Glucose Ketone bodies Lactate (when plasma concentration is elevated) | Lactate |
Skeletal muscle | Glucose Free fatty acids Triacylglycerols Branched-chain amino acids Lactate | Lactate Alanine Glutamine |
Heart | Free fatty acids Triacylglycerols Ketone bodies Glucose Lactate | |
Liver∗ | Amino acids (partial oxidation) Free fatty acids Lactate Glycerol Glucose Alcohol | Glucose Ketone bodies Lactate (during absorptive phase) Triacylglycerols |
Intestine† | Glucose Glutamine | Lactate Alanine |
Red blood cells | Glucose | Lactate |
Kidney | Glucose Free fatty acids Ketone bodies Lactate Glutamine | Glucose (renal gluconeogenesis important in prolonged starvation) |
Adipose tissue | Glucose Triacylglycerols | Glycerol Free fatty acids Lactate |
∗The liver is also the site of galactose and fructose metabolism.
†The small intestine also releases dietary glucose, galactose, fructose, amino acids, and lipids.
In this chapter, fuel utilization in the postprandial or absorptive (fed) state is compared to that in the postabsorptive (fasted) and more extreme starved state. These contrasts illustrate various regulatory processes governing macronutrient metabolism and fuel utilization. A further discussion of fuel utilization by skeletal muscle in the context of rest and exercise is the topic of Chapter 20. In preparation for describing the changes in fuel utilization that occur under these conditions, this chapter begins with a brief overview of the mechanisms used by tissues to regulate metabolism in the face of changing fuel availability or energy need.
Regulation of Macronutrient Metabolism at the Whole-Body Level
Several whole-body mechanisms play an extremely important role in the regulation of fuel metabolism.
Changes in Nutrient Supply
Release of endocrine hormones, particularly the pancreatic hormones insulin and glucagon, responds to meal size and composition and thus plays an over-arching role in communicating the body’s status with respect to influx of nutrients from the gastrointestinal tract to various tissues and in triggering metabolic remodeling of the tissue to respond appropriately to changes in nutrient supply. As discussed in Chapters 12 and 16, high insulin/low glucagon promotes lipid and carbohydrate storage, whereas low insulin/high glucagon results in stimulated adipose tissue lipolysis and hepatic glucose production. High insulin and low glucagon levels are characteristic of the postprandial state, whereas low insulin and high glucagon levels are characteristic of the fasted state.
The body has a complex system for regulation of food intake and energy expenditure that involves the central nervous system’s integration of numerous signals from the gastrointestinal tract, pancreas, and adipose tissue. Output from the central nervous system stimulates changes in food intake to maintain energy balance. These include the impetus to initiate eating and also the satiety/satiation effects that promote the ending of a meal; regulation of food intake is discussed in more detail in Chapter 22.
Underlying the use of macronutrients by tissues in response to changes in nutrient availability is the metabolic flexibility of most tissues to use more than one fuel. Glucose and fatty acids are the major fuels used by tissues, and most tissues can use either glucose or fatty acids. The “glucose–fatty acid cycle,” which was first proposed by Randle and associates (1963), is a helpful concept in understanding fuel flux between tissues and fuel selection by tissues. The primary tenet of the Randle cycle was that the increased provision of exogenous lipid fuels or the increased breakdown of endogenous triacylglycerol stores promotes the use of lipid fuels and, in so doing, blocks the utilization of glucose. This was based on studies with isolated heart and skeletal muscle preparations in which they found that the provision of lipid fuels (fatty acids, ketone bodies) suppressed glucose uptake and glycolysis whether or not hormones were added. Thus the glucose–fatty acid cycle drew attention to the competition between glucose and fatty acids for their oxidation in muscle. Although hormones such as insulin play important roles not only in determining nutrient availability but also in altering the metabolic capacities of tissues, Randle’s observation that the utilization of one nutrient could inhibit the use of the other nutrient directly and without hormonal mediation introduced the concept of nutrient-mediated fine-tuning of fuel utilization that occurs on top of the more coarse substrate availability/hormonal effects. These observations also led to the discovery of biochemical mechanisms by which fatty acids suppressed glucose utilization and, subsequently, of mechanisms by which glucose could suppress fatty acid utilization (Hue and Taegtmeyer, 2009). Some of these mechanisms are discussed in subsequent text and in Chapters 12 and 16.
Distributed Control
Consideration of the many factors that influence fuel utilization has led to the understanding that metabolic regulation of fuel utilization is under “distributed control” by several different processes, and that the steps that limit fuel utilization vary in response to physiological and biochemical changes. Both whole-body and cell-specific processes are involved. An excellent example of distributed control is provided by the work of Wasserman and colleagues on the regulation of glucose flux into muscle in vivo (Wasserman et al., 2011). Muscle glucose uptake is regulated by control of glucose delivery to muscle, control of membrane transport into muscle, and control of the phosphorylation of glucose within muscle. Glucose transport into muscle is regulated by translocation of the insulin-responsive glucose transporter GLUT4 to the plasma membrane. In the fasted state GLUT4 limits glucose uptake, but in the insulin-stimulated or fed state the upregulated GLUT4 glucose uptake is such that other factors become more limiting. Carbohydrate intake and hepatic glucose production are important factors affecting glucose concentrations and hence muscle glucose delivery. Glucose is phosphorylated by hexokinase, which traps glucose in the myocyte. If glucose 6-phosphate accumulates, this feedback inhibits hexokinase and slows glucose uptake and utilization. Pathways downstream of glucose phosphorylation (i.e., glycogen synthesis and glycolysis) can play a role in control of glucose uptake through their effects on glucose 6-phosphate levels.
Regulation of Macronutrient Metabolism at the Cellular Level
Biochemical Mechanisms of Regulation of Metabolic Pathways
Regulation of Flux through a Metabolic Pathway
Although regulation of metabolism generally involves many types of regulation at many different steps in a pathway and related pathways and processes, some changes have large effects on flux through a pathway, while other changes have little or no effect. Obviously, those that result in changes in flux are more significant physiologically, but it is not always easy to discern the consequence of an individual change in the context of the whole body. One example of a highly regulated enzyme with substantial physiological consequences for cholesterol synthesis is 3-hydroxy-3-methylglutaryl-CoA (HMG-CoA) reductase (Goldstein et al., 2006). This is an early step in the overall cholesterol synthetic pathway that is highly regulated and can exhibit a many-fold change in activity. HMG-CoA reductase is the target of the statin drugs used to treat patients with hypercholesterolemia.
Short-Term and Long-Term Regulation
In describing the regulation of the cellular capacity to perform various enzymatic reactions or other processes, we often speak of short-term and long-term regulation. As their names imply, they may be distinguished by the length of time required for bringing about changes, but this distinction does not apply absolutely. In reality, these two terms refer to two distinct mechanisms of regulation. Short-term control refers to changes in the specific activity of an enzyme (or transporter, transcription factor, etc.), with no change in its concentration. Long-term control refers to changes in the amount of enzyme (or transporter, transcription factor, etc.), with no change in its kinetic properties. The two mechanisms are not exclusive, and some proteins are subject to both short-term and long-term control in response to the same stimulus or different stimuli. In some cases, a long-term response can occur as rapidly as a short-term response; control of protein concentration is highly dependent on the half-life of the protein and new steady state concentrations are reached quickly for proteins with very short half-lives (see Chapter 13). Regardless of whether they are short-term or long-term, regulatory mechanisms must be reversible if they are to be of physiological importance. In other words, the concentration or the activity state of the protein must be able to be both upregulated and downregulated.
Short-Term Control of Metabolism
Short-term control can be brought about by a variety of mechanisms. Many specific examples are given in Chapters 12, 14, 16, and 17.
Changes in Levels of Key Metabolites or Regulators
Changes in fuel availability may bring about changes in flux through metabolic pathways. Either an altered concentration of a substrate or an altered concentration of a metabolite in the pathway can result in a change in the concentration of allosteric effectors. Short-term regulation is often related to small changes in the levels of key intermediates such as ATP and NADH. This may result simply from changes in cosubstrate or coenzyme supply. For example, a change in NADH concentration will usually be mirrored by an opposite change in NAD+, and a change in acyl-CoA concentration likewise will result in an opposite change in the free coenzyme A (CoA) concentration. Any change in these ratios will affect flux through enzymatic reactions utilizing one of these couplets. Both allosteric activation and inhibition are also important short-term mechanisms that involve the binding of a regulatory molecule to a distinct site on an enzyme. Several examples of short-term control can be seen in the pathway for glycolysis. For example, ATP is a strong inhibitor of 6-phosphofructo-1-kinase, and this inhibition can be relieved by a second allosteric factor, such as fructose 2,6-bisphosphate in liver or AMP in muscle (see Chapter 12, Figure 12-8). Hexokinase is inhibited by glucose 6-phosphate, restricting uptake of glucose in tissues that do not express the glucokinase isoform, which is insensitive to the inhibitory effects of glucose 6-phosphate. Hepatic pyruvate kinase is stimulated by fructose 1,6-bisphosphate, which is elevated when glucose is being metabolized, and inhibited by alanine, which is an important gluconeogenic substrate during starvation (see Chapter 12, Figure 12-8). In addition, hepatic pyruvate kinase is subject to covalent modification (phosphorylation) by 5′-cyclic adenosine monophosphate (cAMP)-dependent protein kinase A (PKA); this makes it more sensitive to allosteric inhibition. The inhibition of mitochondrial uptake of long-chain fatty acids by the effects of malonyl CoA on the carnitine palmitoyltransferase 1 (CPT1) is another example of regulation by allosteric effects of small molecules; in this case a substrate or intermediate in a competing pathway, fatty acid synthesis, acts to inhibit the opposing pathway, fatty acid oxidation.
Reversible Covalent Modification of Proteins
Reversible covalent modification of proteins is another important short-term regulatory mechanism. A common type of regulatory modification is phosphorylation–dephosphorylation, catalyzed by kinases and phosphatases. Phosphorylation of proteins usually occurs on particular serine, threonine, or tyrosine residues of the proteins, resulting in activation of some proteins and in inhibition of others. Phosphorylation may affect the target protein by changing its kinetic properties, its ability to associate with other proteins, its sensitivity to allosteric effectors, or its intracellular localization. Important examples of enzymes that are controlled by phosphorylation–dephosphorylation include hormone-sensitive lipase in adipose tissue, which is activated by phosphorylation in response to catecholamines and inactivated by dephosphorylation in response to insulin (see Chapter 16, Figure 16-9); acetyl-CoA carboxylase in liver, which is activated by dephosphorylation in response to insulin, so that cytosolic acetyl-CoA is converted to malonyl-CoA (see Chapter 16, Figure 16-3); glycogen synthase, which is inactivated by phosphorylation in response to glucagon in liver (see Chapter 12, Figure 12-15); and 6-phosphofructo-2-kinase, a bifunctional enzyme that loses kinase activity while its fructose 2,6-bisphosphatase activity is enhanced in response to glucagon in liver (see Chapter 12, Figure 12-10).
Another very important phosphorylation is that of the pyruvate dehydrogenase complex, which is inactivated by phosphorylation catalyzed by a specific kinase bound to the enzyme complex. Phosphorylation (inactivation) of the pyruvate dehydrogenase complex is stimulated by acetyl-CoA and NADH and inhibited by high CoA, NAD+, or pyruvate (see Chapter 12, Figure 12-18). These effectors influence the activity of the pyruvate dehydrogenase kinase that catalyzes the covalent modification and inactivation of the pyruvate dehydrogenase complex. The tissue-specific expression of different isoforms of pyruvate dehydrogenase kinase and phosphatase allows different tissues to respond differently in terms of pyruvate dehydrogenase complex inactivation during starvation (Sugden and Holness, 2006; Harris et al., 2002).
Changes in Intracellular Localization of Proteins
A somewhat different form of short-term control is the physical movement of proteins within a cell. Sequestering a protein in a subcellular compartment, which is often facilitated by other proteins that associate with the regulated protein, can remove it from the spatial location where it carries out its function. In contrast, processes that facilitate the movement of a protein to the location where it carries out its function (e.g., the movement of a transcription factor to the nucleus or a transporter to the plasma membrane) will upregulate its physiological effect. A well-known example of this is the sequestering of GLUT4 glucose transporters in intracellular vesicles until insulin activates the movement of these insulin-sensitive glucose transporters to the cell surface of myocytes and adipocytes. The increase in GLUT4 transporters in the plasma membrane increases the glucose transport capacity of these cells and can result in increased uptake and utilization of glucose by the tissue. Sometimes a combination of methods of short-term regulation are involved as, for example, in the phosphorylation of forkhead box class O (FoxO) transcription factors by Akt, which results in exclusion of FoxO from the nucleus where it would act as a transcriptional activator. Another example is the movement and activation of glucokinase and glycogen synthase within the liver in response to hormones, a process that results in these proteins becoming physically close to or removed from their substrates (Watford, 2002). In the postabsorptive state glucokinase is present within the nucleus where it is bound by a glucokinase regulatory protein together with fructose 6-phosphate. During the absorptive period in the presence of fructose 1-phosphate or high glucose, glucokinase is released from the regulatory protein and translocates to the cytosol where it is active in hepatic glucose uptake and metabolism (see Chapter 12, Figure 12-9).
Long-Term Control of Metabolism
An example of a highly regulated key enzyme of hepatic glucose metabolism is phosphoenolpyruvate carboxykinase (PEPCK). This protein has a half-life of about 6 hours and is regulated exclusively by long-term mechanisms. Most of this regulation is brought about by changes in the rate of transcription of the PEPCK gene and hence changes in the overall level of enzyme protein (see Chapter 12, Figure 12-8). The fall in circulating insulin during starvation allows the FoxO transcription factors to enter the nucleus and activate transcription of the PEPCK gene. In addition, the rise in circulating glucagon during starvation acts via cAMP to stimulate transcription of the PEPCK gene. Thus, as starvation slowly develops and as liver glycogen stores are gradually depleted, the liver is synthesizing new PEPCK and thereby increasing its capacity to carry out gluconeogenesis. Such a mechanism is highly suited to a situation such as starvation in which changes in metabolism occur over hours or days. On the other hand, this mechanism would not be suitable for rapid responses such as those needed for muscle contraction, during which short-term regulatory mechanisms are necessary to allow for compensatory responses within fractions of a second.
Long-term changes can improve or diminish short-term regulatory responses to subsequent physiological stressors. An example of how long-term adaptation can affect short-term fuel availability is illustrated by a study in which aerobically trained men were adapted to a 75% fat/5% carbohydrate diet or to a 30% fat/50% carbohydrate control diet (Pehleman et al., 2005). Adaptation to the high-fat/low-carbohydrate diet increased muscle pyruvate dehydrogenase kinase activity, which decreased pyruvate dehydrogenase activation to the “a” form, and decreased the oxidative disposal of glucose by skeletal muscle. Subsequent administration of an oral glucose load to these men resulted in decreases in pyruvate kinase activity and increases in pyruvate dehydrogenase activity, in both subjects fed the control diet and in subjects fed the high-fat/low-carbohydrate diet. However, the absolute level of pyruvate dehydrogenase “a” remained lower in men adapted to the control diet.
Many Proteins are Regulated by Multiple Mechanisms
phosphorylated and converted to a less active form by AMP-activated protein kinase (AMPK). In addition, acetyl-CoA carboxylase is regulated by allosteric effectors. The activity of acetyl-CoA carboxylase is increased by citrate, the cytosolic source of acetyl-CoA substrate that is present when glucose is abundant, and decreased by long-chain fatty acyl-CoAs, which are elevated during active lipolysis and β-oxidation when production of malonyl-CoA and palmitate from glucose would be undesirable. The presence of two genes encoding acetyl-CoA carboxylase 1 and 2 allow tissue-specific regulation of their expression. The first form is predominantly expressed in lipogenic tissues and is cytosolic. The second form is expressed in heart, skeletal muscle, and liver and has a unique N-terminal sequence that targets acetyl-CoA carboxylase 2 for insertion in the mitochondrial membrane. Its location near CTP1 facilitates its role in regulation of fatty acid transport into mitochondria for β-oxidation. Thus in liver of subjects consuming a high-carbohydrate diet, acetyl-CoA carboxylase 1 concentration would be high, and the enzyme would be active due to lack of phosphorylation by AMPK, to allosteric inactivation by citrate, and to lack of inactivation by long-chain acyl-CoAs.
Integrative Pathways for Regulation of Macronutrient Metabolism at the Cellular Level
Role of Amp-Activated Protein Kinase in the Regulation of Cellular ATP Levels


A protein kinase that responds to changes in AMP levels is an important regulator of ATP levels at the cellular level. This kinase is called 5′-AMP-activated protein kinase, usually referred to as AMPK, or somewhat inappropriately as “AMP kinase.” As shown in Figure 19-1, AMPK is phosphorylated by various upstream kinases (AMPKKs such as LKB1 and calmodulin-dependent protein kinase kinase β) and dephosphorylated by a phosphatase. The AMPKKs specifically phosphorylate residue Thr172 of the α-subunit of AMPK. When the energy status of the cell is low, as indicated by a high AMP/ATP ratio, AMPK is in its active phosphorylated state. Phosphorylation of AMPK may be regulated both by activation of an upstream AMPKK, although some of these are thought to be constitutively active, and by the allosteric regulation of the phosphorylation state of AMPK by AMP (Witczak et al., 2008; Carling et al., 2011). The allosteric regulation of AMPK by AMP involves the binding of AMP to the γ-subunit of AMPK; this appears to make AMPK a better substrate for the upstream kinases and a poorer substrate for the phosphatase. Binding of AMP at another site may also activate AMPK directly.
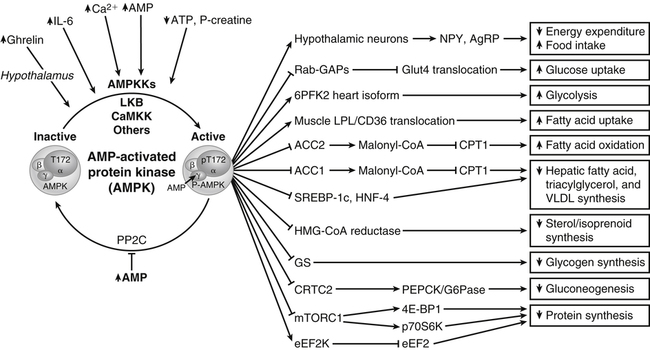
AMP-activated protein kinase (AMPK) is phosphorylated by various kinases (AMPKK) and dephosphorylated by a phosphatase (PP2C) that is inhibited by AMP. When energy status of the cell is low, as indicated by a high AMP/ATP ratio, AMPK is in its active state (phosphorylated at Thr172). AMPK acts on a number of downstream substrates to stimulate processes that lead to increased ATP production and to inhibit processes that lead to increased ATP consumption. The net effect is a return of ATP and AMP levels to the normal range. Specific examples of AMPK targets are shown with — = inhibition/deactivation and ⟶ = stimulation/activation. The direction of the arrows inside of boxes indicates the final effect on the pathway or process listed in the box. Examples include the activation of NPY/AgRP hypothalamic neurons, leading to increased food intake and decreased peripheral energy expenditure; inhibition of Rab GTPase-activating proteins (i.e., Akt substrate 160), thereby permitting GLUT4 translocation to increase glucose uptake in muscle; activation of the heart isoform of 6-phosphofructo-2-kinase (6PF2K), increasing glycolysis; enhanced secretion of lipoprotein lipase (LPL) and enhanced translocation of CD36 fatty acid transporter to plasma membrane, which increases fatty acid uptake by muscle; inhibition of acetyl-CoA carboxylase (ACC2) to prevent malonyl-CoA formation which would block transport of fatty acids into the mitochondria. Other examples include the inhibition of ACC1 in liver, leading to decreased hepatic fatty acid, triacylglycerol and VLDL synthesis; inhibition of SREBP1c and HNF4 transcription factors, which prevents their activation of lipogenic gene transcription; inhibition of cytosolic HMG-CoA reductase, resulting in decreased cholesterol/isoprenoid synthesis; inhibition of glycogen synthase (GS), limiting the storage of glucose as glycogen; inhibition of cAMP element binding protein (CREB) regulated transcription coactivator 2 (CRTC2) which in turn inhibits cAMP response element (CRE)-mediated transcription of gluconeogenic genes; stimulatory phosphorylation of tuberous sclerosis complex 2 (TSC2) or inhibitory phosphorylation of the mammalian target of rapamycin complex 1 (mTORC1), either of which will inhibit mTORC1 activity and lead to inhibition of protein synthesis and activation of autophagy (not shown); and activation of eukaryotic elongation factor 2 kinase (eEF2K) which will lead to inhibition of eEF2 required for protein synthesis.
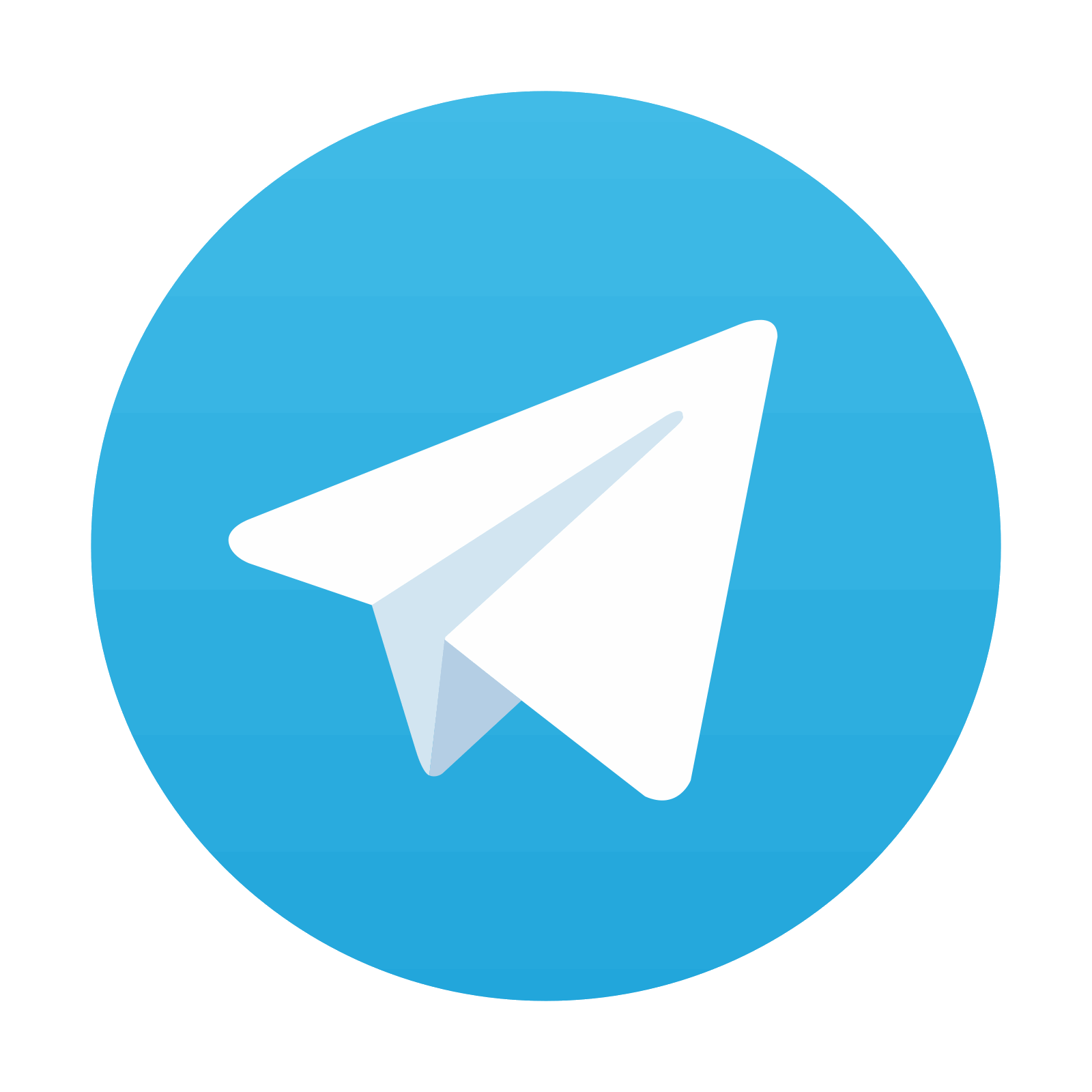
Stay updated, free articles. Join our Telegram channel
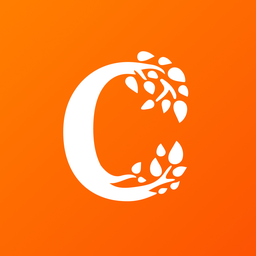
Full access? Get Clinical Tree
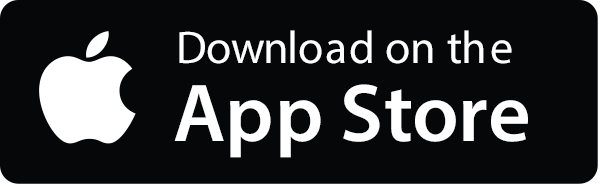
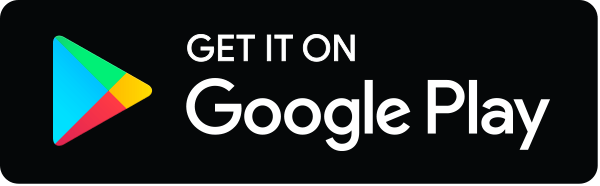