Fig. 6.1
(Top; a): Construction of the characteristic curve for a silicone tube used as a bridge for a gap of variable length in the mouse (left) and rat (right) sciatic nerves. Each curve summarizes data from several independent investigators. Frequency of innervation of the gap, %N, is plotted against the gap length employed by the investigator. The data show a sharp drop in %N at the critical axon elongation, Lc, a characteristic of a given experimental configuration and animal species. Lc is the gap length above which the chance of observing innervation is near zero. Lc for the rat sciatic nerve is estimated at 9.7 ± 1.8 mm; for the mouse sciatic nerve it is 5.4 ± 1.0 mm. The S-shaped curve comprising silicone tube data shown above has been used as a standard for comparison of various tubulated configurations. (Bottom; b): Superposition of data from studies of mouse and rat sciatic nerve. Rat and mouse data were superposed by plotting %N data against the reduced length, L/Lc, the ratio of the gap length at which an observation of %N was made in a rodent species divided by the critical axon elongation for that species.
If sufficient data are available to construct the characteristic curve for a given device, it is possible to estimate the value of L c for the device directly, as follows. Having plotted the entire %N vs. L curve, construct the abscissa at %N = 50 and locate the intersection of the abscissa with the curve. At the intersection point, read the value of L = L c for that device. Thus, define the critical axon elongation as:

In the absence of such complete data, we hypothesize that the characteristic curves of all devices have the same shape, including an inflection point at %N = 50 for the S-shaped curve (see discussion of this hypothesis below). It follows that all such curves differ only by a horizontal shift (translation) along the gap length axis. Suppose that a publication in the literature reports just a single experimental point for a given device. L c for that device is obtained by constructing its hypothetical characteristic curve and reading L c from that curve. The hypothetical curve can be obtained by first plotting the single available experimental point on the %N vs. L coordinate plane. Now construct on that plane the entire characteristic curve for the silicone tube. The next step is translation of the silicone curve along the horizontal axis (right or left) until it intersects the single available experimental point previously located on the %N vs. L coordinate plane. The translated curve is now the hypothetical characteristic curve for the device and L c can be estimated using the procedure described above for the case of a known characteristic curve .
This procedure has a number of limitations. First, it is based on the hypothesis that the characteristic curves of various devices are identically S-shaped and, therefore, differ from the curve for the silicone standard only by a horizontal shift along the gap length axis. With the exception of isolated cases where it was supported by experimental evidence (Spilker 2000), this assumption has not been adequately tested. Further, the value of L c estimated in this manner is often reported as an inequality. This limitation clearly originates from the same feature that makes it valuable: because of the abrupt drop of %N with gap length in the vicinity of L c (Fig. 6.1), it is possible to observe values of %N that lie between 0 and 100 only within a short interval of the gap length. Values of %N observed considerably outside the narrow range of the critical axon elongation can accordingly be reported only as upper or lower bounds .
Once adopted as an estimation method, the procedure described here normalizes data in the literature and has certain powerful consequences. It enables obtaining estimates of L c from a large number of publications in the literature where only a single experimental point (measured %N at a selected L) has been reported. Use of this approximation makes available a large database of independent observations and leads to conclusions about the regenerative activity of devices and the mechanism of regeneration that are worth reporting until the data available in the literature become more complete. A tabulation of values of critical axon elongation estimated from diverse data from independent studies appears in Table 6.1.
Table 6.1
Regenerative activity of several tubulated configurations. (Data from rat sciatic nerve. Regenerative activity of a configuration is expressed in terms of the critical axon elongation, Lc)
Experimental variable X | L c in presence of X, mm | L c in absence of X, mm | Shift length, ∆ L, mma | References |
---|---|---|---|---|
A. Effects of tubulation, insertion of distal stump and ligation of distal tube end | ||||
Collagen tube vs. no tubulation | ≥ 13.4 | ≤ 6.0 | > 7.4 | Chamberlain et al. 2000a |
Distal stump inserted vs. open-ended tube | 11.7 | ≤ 6.0 | > 5.7 | Williams et al. 1984 |
Distal stump inserted vs. ligated distal end | 11.7 | ≤ 6.0 | > 5.7 | Williams et al. 1984 |
B. Tube wall composition | ||||
Silicone tube (standard) | 9.7 | (9.7)b | 0 | Data in Fig. 6.1 |
EVA copolymerc vs. silicone standard | ≤ 11.0 | (9.7)b | ≤ 1.3 | Aebischer et al. 1989b |
PLA, plasticizedd vs. silicone standard | ≥ 13.4 | (9.7)b | ≥ 3.7 | Seckel et al. 1984 |
LA/ε-CPLe vs. silicone standard | ≥ 13.4 | (9.7)b | ≥ 3.7 | Dunnen et al. 1993b |
Collagen tube vs. silicone tube | ≥ 13.4 | 8.0 | ≥ 5.4 | Chamberlain et al. 1998b |
C. Tube wall permeability | ||||
Cell-permeability vs. impermeability | ≥ 19.4 | 13.4 | ≥ 6.0 | Jenq and Coggeshall 1987 |
Cell-permeability vs. protein-permeability | ≥ 11.4 | 13.4 | ≥ 3.9 | Jenq et al. 1987 |
Protein-permeability vs. impermeability | 7.5 | 8.9 | − 1.4 | Jenq and Coggeshall 1985a; Jenq et al. 1987 |
D. Schwann cell suspensions | ||||
Schwann cell suspension vs. PBS | 21.4 | ≤ 14.0 | ≥ 7.4 | Ansselin et al. 1997 |
E. Tube filling: solutions of proteins | ||||
bFGF vs. no factor | 14.3 | ≤ 11.0 | > 3.3 | Aebischer et al. 1989b |
NGF vs. cyt Cf | 11.1 | 10.4 | 0.7 | Hollowell et al. 1990 |
aFGF vs. no factor | 15.5 | ≤ 11.0 | ≥ 4.5 | Walter et al. 1993 |
F. Tube filling: gels based on ECM components | ||||
Fibronectin vs. cyt Cf | 19.1 | 18.6 | 0.5 | Bailey et al. 1993 |
Laminin vs. cyt Cf | 18.6 | 18.6 | 0 | Bailey et al. 1993 |
G. Tube filling: insoluble substrates | ||||
Collagen-GAG matrix vs. no matrix | 16.1 | ≤ 11.0 | ≥ 5.1 | Orgill 1985; Yannas et al. 1985, 1987a, c |
Oriented fibrin matrix across gap vs. oriented matrix only adjacent to each stump | 15.5 | ≤ 11. 0 | ≥ 4.5 | Williams et al. 1987 |
Early-forming vs. late-forming fibrin matrix | 15.5 | 12.5 | 3.0 | Williams et al. 1987 |
Axially vs. randomly oriented fibrin | 11.4 | ≤ 6.7 | ≥ 4.7 | Williams 1987 |
Rapidly degrading CG matrix (NRTi) vs. no CG matrix | ≥ 13.4 | 8.5 | ≥ 4.9 | Yannas et al. 1988b; Chang et al. 1990; Chang and Yannas 1992 |
Axial vs. radial orientation of pore channels in NRTi | ≥ 13.4 | 10.0 | ≥ 3.4 | Chang et al. 1990; Chang and Yannas 1992 |
Laminin-coated collagen sponge vs. no laminin coating | 11.7 | ≤ 6.0 | > 5.7 | Ohbayashi et al. 1996 |
Polyamide filamentshvs. no filaments | 18.4 | ≤ 11.0 | ≥ 7.4 | Lundborg et al. 1997 |
NRTg in collagen tube vs. silicone tubei | > 25 | 7.7 | > 17.3 | Spilker 2000 |
We now examine data that were obtained with different species. An analysis similar to the one described above for the rat leads to the conclusion that mouse sciatic nerve data from several independent investigators who studied the silicone tube configuration can also be used to construct a standard curve for the mouse (Fig. 6.1a; top, left curve). The mouse data are consistent with a significantly lower value for critical axon elongation than for the rat, namely, L c = 5.4 ± 1.0 mm. Rat and mouse data were superposed simply by plotting %N data against the reduced length, L/L c , the ratio of the gap length, L, at which an observation of %N was made in a rodent species, divided by the critical axon elongation, L c , for that species. We observe that the data superpose (Fig. 6.1; bottom). The ability to superpose data from two species suggests that the relation between frequency of reinnervation and gap length reflects a general phenomenon that may not be species-specific .
Data obtained with different species show large differences (Fig. 6.1; bottom). The data show that the critical axon elongation for the mouse sciatic nerve, L c = 5.4 ± 1.0 mm, is significantly lower than that for the rat sciatic nerve, L c = 9.7 ± 1.8. This finding immediately questions in a quantitative fashion the practice of comparing the effectiveness of nerve chambers that were studied with a variety of animal species by simply citing the absolute gap length along which regeneration had been observed. Clearly, a report of an absolute gap length, frequently encountered in the literature, is not meaningful by itself. Data from different animals can only be compared directly if the disparate anatomical lengths have been normalized.
There is a further consequence. Division of the gap length, L, at which an observation of %N was made for a given species, by the critical axon elongation , L c , for the same species, to yield the reduced gap length, L/L c , we obtain a single S-shaped relation between %N and gap length for both species (Fig. 6.1; bottom). The superposition of data achieved in this manner describes the behavior of the device from both mouse and rat in a single reduced curve. Although detailed data with other species were not available when this review was prepared, it is suggested that this simple scaling law may extend to other species as well. For example, it might be possible in future studies to compare quantitatively, regeneration data obtained with a given device in the rat at a 20-mm gap length and that conducted in a monkey or a human across a 50-mm gap. Such a potential correlation would be very valuable.
6.1.5 Long-Term Assays (> 20 weeks): Fidelity of Regeneration
Having described above an assay for the self-consistent evaluation of tubulated configurations using short-term data (typically < 20 weeks), we now proceed to a selection of suitable long-term assays. These assays report the extent to which the regenerate has achieved physiological function, as shown by a comparison of functional data for the regenerate with normal values.
Long-term assays are intuitively based on the concept of fidelity of regeneration, defined simply as the fractional value of a property Y of the regenerate relative to its normal (physiological) value, as follows: Fidelity of regeneration (% FR) = (value of property Y in regenerate/value of property Y in normal organ). Properties Y have included those based on both morphological and functional assays and are presented in Table 6.2.
Table 6.2
Long-term (> 20 weeks) studies of induced regeneration of peripheral nerves in experimental animals. Electrophysiological data. (Expressed as fraction of normal value (fidelity of regeneration))
Experimental parameters | Electrophysiological properties (fraction of normal value) | ||||||
---|---|---|---|---|---|---|---|
Animal model (length of study, weeks) | Gap length, mm | Tube | Tube filling | Latency | Conduction velocity | Amplitude | References |
Sciatic, rat (43) | 10 | Silicone | None | (1) | 0.64 | 0.09 | Fields and Ellisman 1986a; Fields et al. 1989 |
Sciatic, rat (40) | 10 | Silicone | NRTa: 5 μm pores 10 μm 60 μm 300 μm | 1.38 1.39 1.51 1.64 | 0.59 0.63 0.57 0.54 | 0.22 0.55 0.42 0.20 | Chang et al. 1990; Chang and Yannas 1992 |
Sciatic, rat (60) | 10 | Silicone | NRTa: 35 μm | 0.48 | 0.27 | Chamberlain et al. 1998b | |
Sciatic, mouse (21.4) | 6 | Silicone | None | 0.07 | Navarro et al. 1996 | ||
Sciatic, mouse (21.4) | 6 | PTFEb | None | 0.02 | Navarro et al. 1996 | ||
Rabbit, tibial (36) | 10 | PGLc | None | (1) | Molander et al. 1983 | ||
Monkey, median (52) | < 1 | PGAd | None | 0.94 | 0.32 | Tountas et al. 1993 | |
Mouse, sciatic (21.4) | 6 | PLLA/PCLe | None | 0.18 | Navarro et al. 1996 | ||
Monkey, median (200) | 5 | Collagen | None | 1.40 | 0.93 | 0.91 | Archibald et al. 1995 |
Rat, sciatic (60) | 10 | Collagen | None | 0.60 | 0.27 | Chamberlain et al. 1998b | |
Rat, sciatic (60) | 10 | Collagen | NRT: 35 μm pores | 0.75 | 0.36 | Chamberlain et al. 1998b | |
Mouse, sciatic (21.4) | 6 | collagen | None | 0.07 | Navarro et al. 1996 | ||
Cat, sciatic (69) | 25 | PGAd mesh/collagen | NGF/bFGF/laminin | 1.7 | < 1 | (ca. 1) | Kiyotani et al. 1996 |
6.2 Synthesis of Myelinated Nerve Fibers
Having considered problems of uniform presentation of results from assays reported by different investigators, we now turn to the description of the processes that lead to synthesis of peripheral nerves and their tissue components. First we focus on the synthesis of nerve fibers, the simplest functional unit of a nerve, and the tissue immediately adjacent to them, the endoneurium .
For many experimental animals a nerve trunk can be simply viewed as a tissue cylinder, ranging in diameter from a fraction of 1 mm to several millimeters, embedded in which are thousands of tiny cylinders, each with a diameter typically about 20–50 μm. The specialized function of a nerve, electrical conduction, takes place along these tiny cylinders, often referred to as nerve fibers or endoneurial tubes, each comprising a myelinated axon invested in a myelin sheath that, in turn, is ensheathed in a tubular basement membrane (BM) . The tubes are surrounded by a specialized assembly of collagen fibers, the endoneurial stroma. Below we further review the structure of these elementary conducting units.
6.2.1 Structure of Myelinated and Nonmyelinated Axons, and of the Endoneurium
Axons, the long processes of neuronal cells (neurons), are enveloped by SCs, the specialized cells of the nervous system. Both in the intact PNS and in a regenerating nerve, axons can be classified into those that are surrounded by a myelin sheath (myelinated) and those that are nonmyelinated. Axons comprise axoplasm that contains smooth endoplasmic reticulum and microtubules. Whether myelinated or not, axons are embedded in the endoneurium , consisting of a delicate packing of loose vascular supporting tissue that includes collagen fibers (Young et al. 2006; Kierszenbaum and Tres 2012). Several axons with their associated endoneurium are enclosed in a condensed layer of collagenous tissue, the perineurium, and the enclosed bundle of axons comprises a fascicle. Many peripheral nerves consist of more than one fascicle; in these nerves, fascicles are bound together by a collagenous tissue, the epineurium (Young et al. 2006). Each of these three major compartments of a peripheral nerve that are bounded by endoneurium, perineurium, and epineurium, will be described below in the context of data related to their synthesis. Myelinated axons are characterized by a diameter in the range 1–15 μm, and function as efficient conductors of signals that reach target organs, including muscles (motor axons) and sensory organs (sensory axons). During development, myelin is synthesized around axons whose diameter is greater than 0.7 μm, in response to a signal that includes a diffusible molecule (Garbay et al. 2000); furthermore, myelin synthesis is upregulated by contact between adjacent SC plasma membranes (Sasagasako et al. 1999). Differentiation of a SC into a myelinating cell requires simultaneous interactions with BM and an axon destined for myelination (Fernandez-Valle et al. 1997). The myelination of SCs following injury is under study (Zhu et al. 2013; Yao et al. 2014).
The myelination of an individual axon is provided along its length by several SCs. Each cell covers a segment along the axon length (internode); there are points located between successive SCs that are not covered by myelin (nodes of Ranvier). Myelination accelerates conduction by preventing propagation of the action potential along the axon, instead forcing the potential to travel by jumping from node to node (salutatory conduction). The myelin sheath consists of the plasma membrane of the SC that is tightly wrapped around the axon perimeter at the internode; these concentric layers are free of SC cytoplasm. SCs wrapped around an individual axon are ensheathed in a tubular BM that forms a continuous cover of the myelinated axon, even at the nodes. During the process of myelination, concentric layers of SC cytoplasm and plasma membrane envelop the axon; the cytoplasm is then excluded and the inner surfaces of plasma membrane fuse with each other, providing a multiplicity of membrane leaflets that comprise the mature myelin sheath.
The BM surrounding a myelinated axon is very similar to that in skin but is tubular in shape rather than being primarily a flat surface with indentations (rete ridges), as in skin. SC BM contains type IV collagen, laminin, type V collagen, entactin, heparan sulfate proteoglycan, and fibronectin (Bunge and Bunge 1983; McGarvey et al. 1984; Baron-Van Evercooren et al. 1986; Olsson 1990; Obremski and Bunge 1995). Type IV collagen comprises the fine collagen meshwork that is exclusive to BMs in various organs. Laminin binds to type IV collagen via entactin; it also binds to other macromolecular constituents of the BM.
Nonmyelinated axons function in the autonomic nervous system and in small pain nerves. They comprise fibers of relatively small diameter, typically less than 1 mm. These axons are also surrounded by SCs. Unlike myelinated axons, however, the supporting SCs have retained their cytoplasm; they ensheathe the axon but are not wrapped tightly several times around it, and myelin is also absent. Several nonmyelinating axons are frequently surrounded by a single SC (ensheathment) while the external surface of the latter is encased in a BM (Young et al. 2006). Below we focus on myelinating axons.
Using standardized nomenclature, we describe a nerve fascicle as being surrounded by a perineurial sheath that forms the border between the endoneurium inside and the epineurium outside (Olsson 1990). Both myelinated and unmyelinated axons are enclosed in the space inside the fascicle (intrafascicular space) that is bounded by the perineurial sheath (see below). Nerve fibers are closely surrounded by the endoneurial stroma (Fig. 6.2; top), an extracellular matrix resembling a fiber-reinforced gel, comprising collagens, fibronectins, proteoglycans, and other macromolecular components, as well as fibroblasts, macrophages, and mast cells (Olsson 1990). Collagen fibrils in the intrafascicular space comprise both collagens type I and III and have diameters averaging 50 nm (Thomas and Jones 1967; Salonen et al. 1987a, b); following detailed study, a value of 56.1 ± 6.9 nm was also reported (Bradley et al. 1998). The three-dimensional architecture of collagen fibrils surrounding nerve fibers has been shown to consist of two distinct sheaths: The outer one comprises bundles of longitudinally oriented collagen fibrils whereas the inner sheath is a delicate network of interwoven collagen fibrils. Some of the collagen fibrils forming the inner network are closely attached to the BM of SCs. The entire architectural arrangement suggests that the two collagen sheaths serve to tether and position the nerve fibers inside the intrafascicular compartment (Ushiki and Ide 1986).

Fig. 6.2
Structures of physiological endoneurium and perineurium. (Top; a): Diagram suggesting the main features of the endoneurial stroma surrounding nerve fibers. Each nerve fiber (NF) is embedded in a matrix composed of fluid, collagen fibers of the endoneurial stroma, and cells forming the endoneurial microenvironment. The inner surface of the perineurium is shown on the left. (From Olsson 1990) (Bottom; b): Cross sectional view of the perineurium (pn.) of rat sural nerve. Collagen fibers comprising the epineurium (ep.) and endoneurium (en.) are also shown. Bar: 1 µm. (From Thomas and Jones 1967)
The intrafascicular space receives fluid components from the endoneurial blood vessels. The fluid comprises water, various ions and plasma proteins, as well as other soluble compounds. It is maintained under a small but finite hydrostatic pressure; this arrangement stands in contrast to other connective tissue-rich compartments, such as subcutaneous tissue, where a net pressure does not appear to exist. Numerous vessels pierce the perineurium and join the endoneurial vascular network. The endothelial cells of endoneurial vessels are thin, contain vesicles that may be involved in transport phenomena across the endothelium, and are bound by tight junctions. Junctions between the cells contribute to a permeability barrier that protects the space immediately outside the nerve fibers from leakage of certain substances inside the endoneurial blood vessels (blood–nerve barrier, similar but much less restrictive than the blood–brain barrier). The maintenance of a constant environment around the nerve fibers provides protection from changes in ionic strength or from other substances (pathogens) that can modify the conductivity of nerve fibers or otherwise injure them (Olsson 1990).
The nerve trunk can be viewed as comprising two separate vascularized compartments, the epineurium and the endoneurium , kept separated by the permeability barrier of the perineurium (Fig. 6.2, bottom; see below). Testing procedures, based on the selective permeability of these compartments to standard tracers, can be used to detect the presence of endoneurial blood vessels with tight junctions (Azzam et al. 1991). Soon after intravenous injection of albumin or horseradish peroxides, the epineurium shows signs of substantial leakage from blood vessels in it (extravasation), while the mass of tracer extravasated from the endoneurium is much smaller. Thus, the endoneurial environment is protected from various pathogens by both the perineurial barrier and the barrier provided by its own endoneurial blood vessels (Olsson 1990; Azzam et al. 1991).
Advances in understanding the dynamics regulating the exchange of material between the endoneurial microenvironment and the surrounding extracellular space suggest that it may be more accurately described as a blood–nerve interface rather than a blood–nerve barrier. Input to and output from the endoneurial microenvironment occurs via blood–nerve exchange and convective endoneurial fluid flow driven by a proximo-distal hydrostatic pressure gradient (Mizisin and Weerasuriya 2011).
6.2.2 Synthetic Pathways to Myelinated Axons and Basement Membrane
Myelinated axons with their associated BM have been synthesized both in vitro and in vivo.
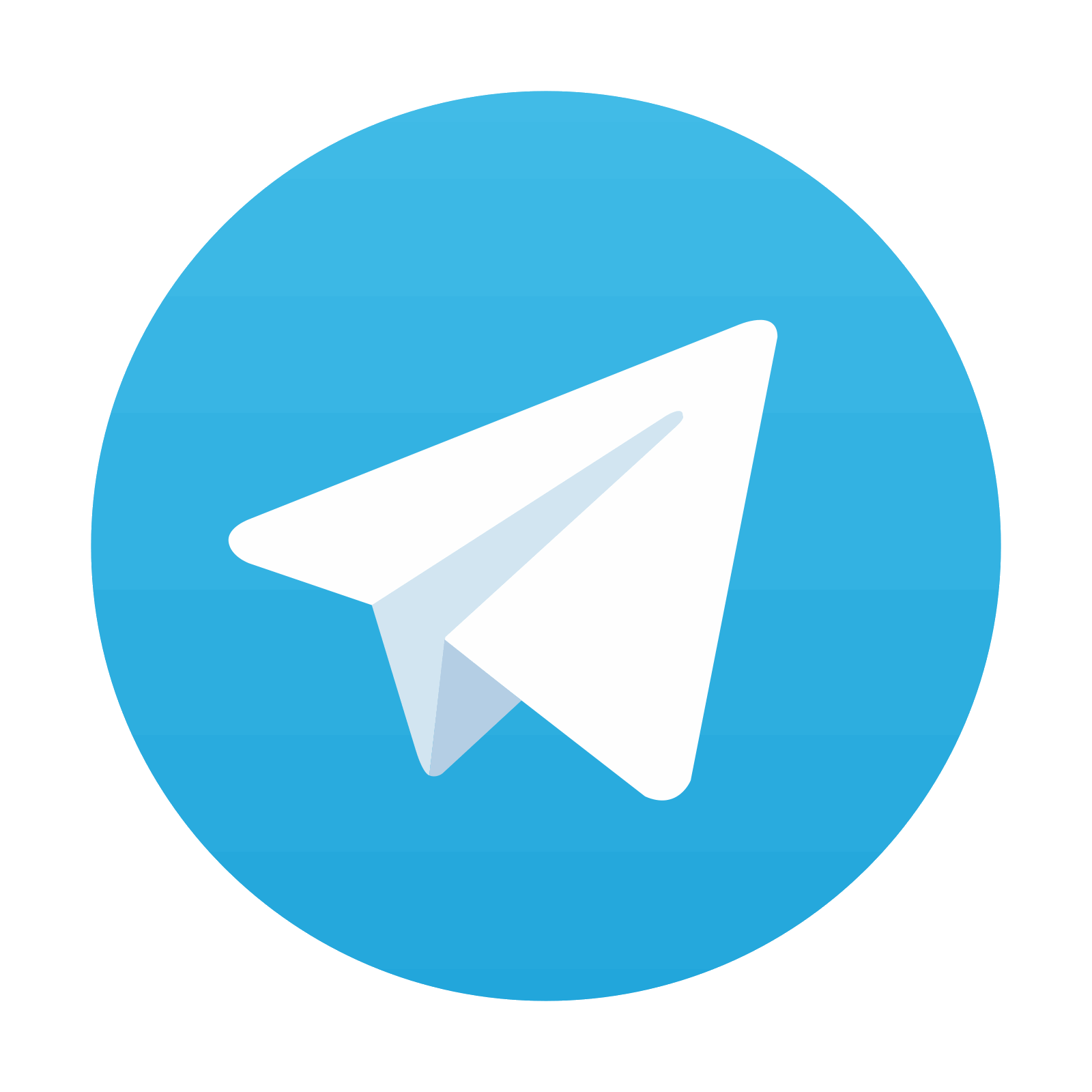
Stay updated, free articles. Join our Telegram channel
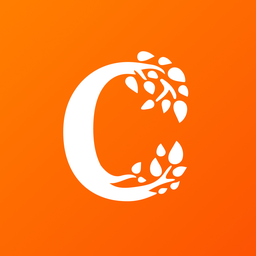
Full access? Get Clinical Tree
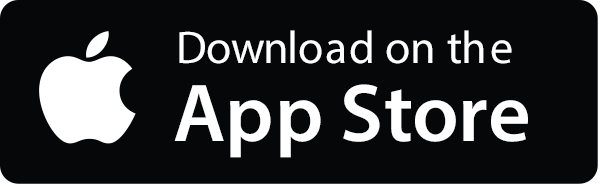
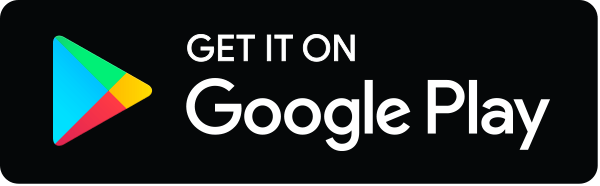