Protein
Method
Units
Collagen scaffold tube
Silicone tube
TGFβ1
ELISA
ng protein per g tissue
43.6 ± 1.8
65.2 ± 4.9
TGFβ1
WB
a.u.
1.29 ± 0.34
1.78 ± 0.66
TGFβ2
WB
a.u.
0.78 ± 0.26
0.93 ± 0.37
TGFβ3
WB
a.u.
3.17 ± 0.53
1.00 ± 0.22
αSMA
WB, IF
a.u.
0.15 ± 0.03
1.13 ± 0.37
The observed downregulation in TGFβ1 concentration in the presence of DRT could result from at least two unrelated sources. There is evidence that TGFβ1 is bound nonspecifically but with great affinity on the DRT surface, to an extent of about 7 µg TGFβ1/mg DRT (Thies 2010). This result raises the question whether the bound TGFβ1 is an equally active contributor to the differentiation of myofibroblasts as is the free growth factor. There is also evidence by electron microscopy that the acetic acid-treated collagen used to fabricate DRT lacks a fraction of its native banding. Loss of collagen banding, without loss of the triple helical structure of collagen, occurs during processing of the scaffold, following swelling in acetic acid solution which is known to selectively melt most of the banding periodicity of collagen fibers at pH levels below 4.25 ± 0.30 (Sylvester et al. 1989). Lack of banding in collagen fibers has, in turn, been associated (Sylvester et al. 1989) with downregulation of the normal collagen-platelet reaction that eventually leads to degranulation (Baumgartner et al. 1976; Jang et al. 2014) and release of TGFβ1 during wound healing. In the absence of platelet degranulation, it is accordingly expected that the TGFβ1 concentration in the wound would be reduced. Although there are unsettled questions about the observed reduction in TGFβ1 concentration in the presence of DRT, its reduction in DRT-treated wounds has been confirmed repeatedly. The consequences of its reduction will be examined below.
Dispersion of assemblies of contractile cells is also observed in the presence of DRT, both in skin wounds and peripheral nerve wounds (Fig. 9.1). A partial explanation can be obtained by comparing the effects on the morphology of contractile cells inside two collagen scaffolds from the same library (Fig. 9.2). The two scaffolds were identical in structure except for the average pore size: DRT had a pore size of 40 µm while the second scaffold (previously referred to as “analog B”) had a pore size of 400 µm (Yannas 2001f). The pore size of the DRT scaffold was within the active range of 20–125 µm, where delay of contraction is significant (Yannas et al. 1989), while analog B did not delay contraction significantly and was considered inactive (Fig. 9.3). The histologic evidence shows that the cell density inside the pores of inactive analog B was much higher than in the pores of active DRT scaffold (cells, mostly fibroblasts, were not stained for αSMA in this study). Furthermore, the large pores in analog B contained thick clusters of cells, approximately 30–50 cells per pore cross section, while the much smaller pores of DRT contained only 2–5 cells per pore cross section (Yannas 2001f). The overall lower cell content of the DRT scaffold can be explained in terms of the lower cell density that characterizes wounds grafted with DRT. Furthermore, cell-scaffold binding would be expected to be much more extensive than cell–cell binding inside DRT due to a purely physical reason; the active scaffold had a lower pore size, corresponding to a higher specific surface, relative to analog B (estimated 12-fold higher specific surface in DRT than in analog B; see Yannas 2001f).

Fig. 9.1
Immunohistochemical localization of αSMA in the contractile cell capsule in skin wounds (top row, red, 10 days post injury) and peripheral nerve wounds (bottom row, brown, gap midpoint, 7 days post injury). Left column: control wounds (top left: ungrafted skin; bottom left: nerve grafted with silicone tube). Right column: wounds grafted with active collagen scaffolds. Arrows: scaffold struts. Scale bars: 100 µm. MFB myofibroblasts

Fig. 9.2
Fibroblasts inside two collagen scaffolds at the center of a skin defect in the guinea pig, day 7. Left: View of highly dense, clustered cells inside the large pores of an inactive scaffold with pore size about 400 µm, specific surface ca. 2000 mm2/mm3 Arrows: scaffold strats. Right: Isolated cells at very low density inside the small pores of an active scaffold, pore size about 40 µm, specific surface ca. 25,000 mm2/mm3. F fibroblasts

Fig. 9.3
Identification of the dermis regeneration template (DRT). The average pore diameter of a collagen scaffold profoundly affects its ability to block wound contraction and its regenerative activity. Full-thickness excisional skin defects in the guinea pig were treated with several scaffolds, differing in pore diameter but otherwise identical in structure that were members of a collagen library. The delay in inset of defect contraction is shown on the vertical axis. The horizontal axis is logarithmic to accommodate the wide range in pore size in this study. DRT is defined within the range 20 to 125 µm where contraction delay is maximized and regeneration occurs. (Yannas et al. 1989)
The isolated presence of cells inside DRT shows that the assemblies of myofibroblasts, based on intercellular adherens junctions (AJs), as described above for normally contracting skin wounds (Hinz et al. 2004), were dispersed in the presence of DRT. Assemblies of contractile cells appear to scale up the contractile force of individual cells to the macroscopic contractile forces that deform wound tissues and eventually close the wound. Such deformation was prominent in the presence of the regeneratively inactive analog B, where contraction takes place almost unchanged from the ungrafted control, but not in the presence of DRT where contraction was blocked (Yannas et al. 1989; Troxel and Yannas 1991; Yannas 1998). Independent studies of the forces that maintain contractile cells assembled during normal contraction suggest that cell–cell contact and consequent coordination among myofibroblasts are required for generation of mechanical forces between cells in normally contracting wounds (Follonnier et al. 2008; Follonier et al. 2010; Goodbout et al. 2013).
Disorientation of long axes of contractile cells present inside the pores of the DRT scaffold is clearly evident in photographs of myofibroblasts in skin wounds and nerve wounds (Fig. 9.1). Such disorientation stands in contrast to the high orientation observed in the absence of DRT, where long cell axes are oriented in the plane of the epidermis in skin wounds or circumferentially around the stumps of transected nerves (Fig. 8.5; Troxel and Yannas 1991; Chamberlain et al. 2000a). The resulting randomization of force vectors contributed by individual cells is expected to lead to extensive mutual cancellation of vectors, leading to reduction of the resultant macroscopic force.
Binding of contractile cells on the DRT surface is the simplest explanation for dispersion of cell assemblies and disorientation of long axes of cells in the presence of DRT. These morphological changes in contractile cells are not observed in the presence of very similar collagen scaffolds used as controls or in the absence of DRT, suggesting that DRT possesses molecular features that distinguish it from closely related controls. Direct evidence of close approach and contact of contractile cells to the scaffold surface has been obtained by transmission electron microscopy (Fig. 9.4; Murphy et al. 1990); however, such evidence at the ultrastructural scale contains no molecular evidence that confirms cell–matrix binding.

Fig. 9.4
Ultrastuctural view of cell-scaffold interactions 7 days after grafting of a full thickness skin defect in the guinea pig with DRT. a Mononuclear cell (not identified further) exhibits pseudopods (black arrow) and contact with the DRT surface. b Mononuclear cell exhibits pseudopods (black arrows) and contact with the DRT surface. c Mononuclear cells (M) formed a contiguous monolayer along the surface of DRT. Magnification: a × 5500. b × 14,000. c × 4500 (Murphy et al. 1990)
What is the molecular evidence that binding between cells and DRT actually takes place? This question will be answered below.
9.2 Specific Binding of Contractile Cells to Ligands on the Surface of DRT
The most abundant isoform of collagen is type I, organized into supramolecular structures (fibrils, fibers) in a manner that depends on the tissue in which it is present (e.g., tendons, dermis, endoneurium, cornea) and regulated by other components of the extracellular matrix (ECM; Gelse et al. 2003; Canty et al. 2005). Collagen microfibrils (pentamers) comprise collagen molecules arranged in groups of five that become organized into much thicker fibrils and fibers, ranging in diameter between 25 and 400 nm. Thicker collagen fibrils belong to a higher structural order than the pentamer (Yannas 1990); they are characterized by a semicrystalline structure identified by a 67-nm periodicity, commonly referred to as the collagen banding pattern. Type I collagen from a variety of animal tissues has been purified and further processed for use as a biomaterial or for in vitro assays. Detailed protocols for preparation of medical-grade devices based on collagen scaffolds for use in regeneration of skin and peripheral nerves have been published (Chamberlain and Yannas 1998) See Appendix.
Collagen adhesion receptors participate in binding various types of mammalian cells on the surface of collagen fibers. The most important of such adhesion receptors are members of the integrin family (Barczyk et al. 2010; Leitinger 2011). Four of these integrins (α1β1, α2β1, α10β1, α11β1) comprise the family of collagen-binding integrins (CBI; Hynes 2002). The specificities of the four CBIs for collagens occasionally overlap or even play contrasting roles (Znoyko et al. 2006). Quite importantly, integrin expression depends on cell type. The α1β1 is abundant in smooth muscle cells, mesenchymal cells, hepatic stellate cells, pericytes, bone marrow stem cells, chondrocytes, and neuronal cells, while α2β1 is expressed in epithelia, lymphocytes, and platelets (Popova et al. 2007; Hamaia et al. 2012). There are also several types of nonintegrin collagen receptors (Leitinger 2011).
Integrins consist of two subunits, referred to as α and β, each subunit comprising four parts: head, leg, transmembrane section, and cytoplasmic domain. The α subunits of CBI include an additional domain close to their N terminus, usually called “I domain” (occasionally referred to as “A domain”). Binding of an integrin on a ligand appears to be mediated entirely by the I domain of the integrin (Hynes et al. 2002; Luo et al. 2007). The I domains have been used as markers of adhesion ligands of the parent integrin, providing means for assay of ligands on DRT (Tzeranis 2013). Integrins transmit chemical information in two directions (bidirectional signaling; Hynes 2002). In “outside-in” signaling, occurring during binding of an integrin to an adhesion ligand located in the extracellular matrix (ECM), the signal from the ECM-binding event is transmitted to that part of the integrin molecule which is located inside the cell (cytosolic domain) through conformational changes and activates downstream signal pathways. In “inside-out” signaling, the adhesiveness is dynamically regulated. Bidirectional signaling is considered essential for rapid response of the cell to environmental changes (Hu and Luo 2013).
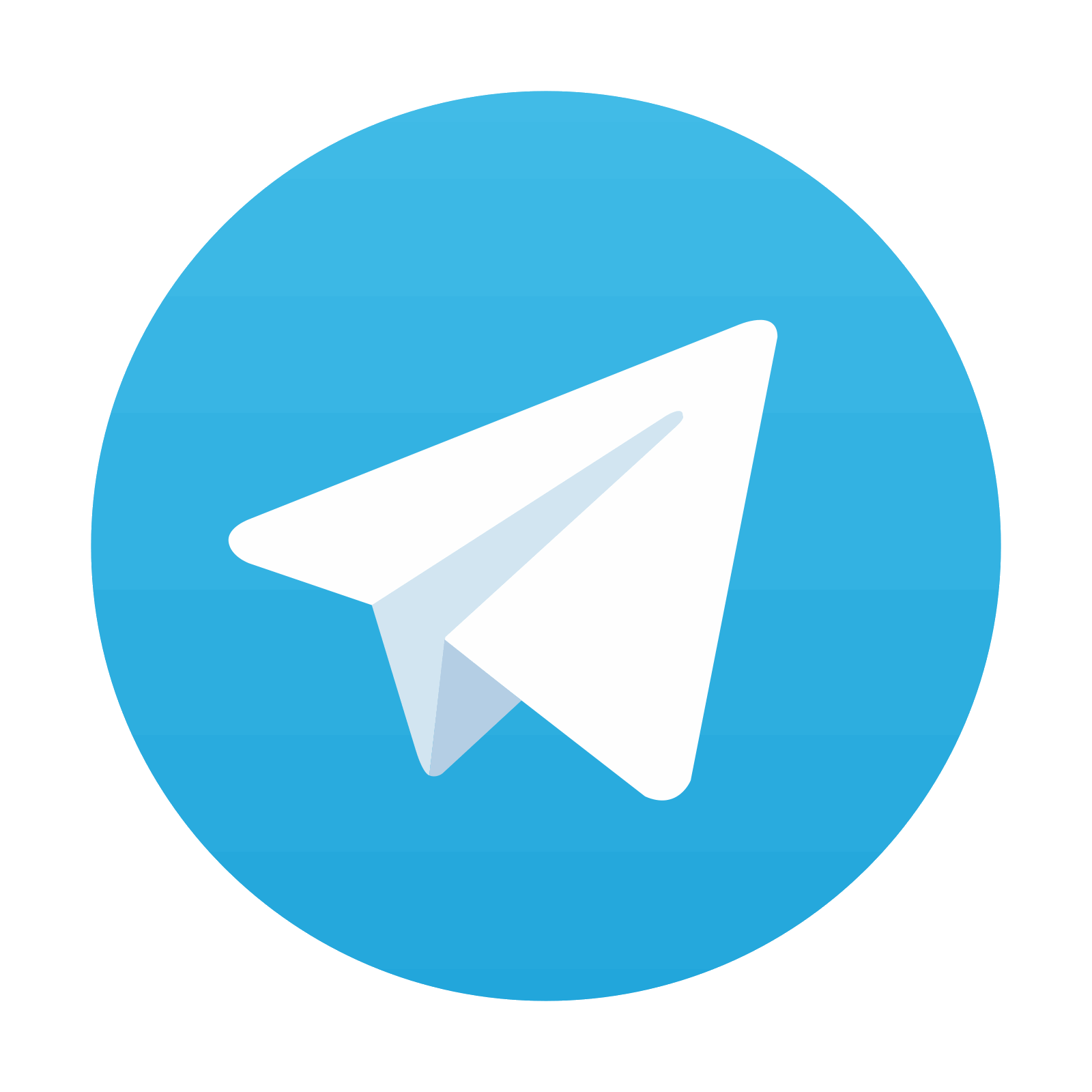
Stay updated, free articles. Join our Telegram channel
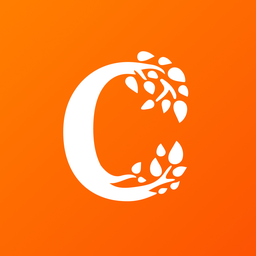
Full access? Get Clinical Tree
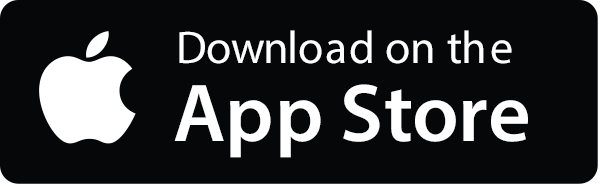
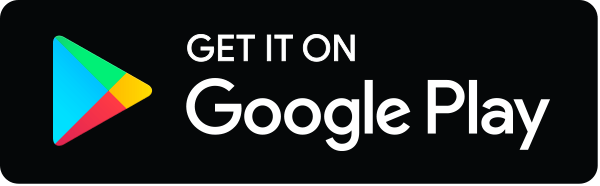