52
Red & White Blood Cells
OBJECTIVES
After studying this chapter, you should be able to:
Understand the concept of stem cells and their importance.
Summarize the causes of the major disorders affecting red blood cells.
Discuss the general structure of the red blood cell membrane.
Know the biochemical bases of the ABO blood group substances.
Indicate the major biochemical features of neutrophils and understand the basis of chronic granulomatous disease.
Appreciate the importance of integrins in health and disease.
BIOMEDICAL IMPORTANCE
Blood cells have been studied intensively because they are obtained easily, because of their functional importance, and because of their involvement in many disease processes. The structure and function of hemoglobin, the porphyrias, jaundice, and aspects of iron metabolism are discussed in previous chapters. Table 52-1 summarizes the causes of a number of important diseases affecting red blood cells; some are discussed in this chapter, and the remainder are discussed elsewhere in this text. Anemia is a very prevalent condition with many causes. The discovery of the causes of certain types of anemias (eg, of pernicious anemia [a form of B12 deficient anemia] and of sickle cell anemia) has been an area where the reciprocal relationship between medicine and biochemistry referred to in Chapter 1 has been extremely beneficial. The World Health Organization (WHO) defines anemia as a hemoglobin level of <130 g/L in men and <120 g/L in females. There are many causes of anemia; only the most prevalent or biochemically relevant are mentioned here. A simplified classification of the causes of anemia is given in Table 52-2. It has been estimated that some 300,000 children are born each year with a severe inherited disorder of hemoglobin, the majority in low- or middle-income countries. Because infant mortality is decreasing, many of these children will survive to present a global health problem. Certain of the blood group systems, present on the membranes of erythrocytes and other blood cells, are of extreme importance in relation to blood transfusion and tissue transplantation. Every organ in the body can be affected by inflammation; neutrophils play a central role in acute inflammation, and other white blood cells, such as lymphocytes, play important roles in chronic inflammation. Leukemias, defined as malignant neoplasms of blood-forming tissues, can affect precursor cells of any of the major classes of white blood cells; common types are acute and chronic myelocytic leukemia, affecting precursors of the neutrophils; and acute and chronic lymphocytic leukemias. Knowledge of the molecular mechanisms involved in the causation of the leukemias is increasing rapidly, but is not discussed in any detail in this text. Combination chemotherapy, using combinations of various chemotherapeutic agents, all of which act at one or more biochemical loci, has been remarkably effective in the treatment of certain of these types of leukemias. Understanding the role of red and white cells in health and disease requires a knowledge of certain fundamental aspects of their biochemistry.
TABLE 52–1 Summary of the Causes of Some Important Disorders Affecting Red Blood Cells
TABLE 52–2 A Brief Classification of the Causes of Anemia
ALL BLOOD CELLS DERIVE FROM HEMATOPOIETIC STEM CELLS
Figure 52–1 summarizes the derivation of the various types of blood cells from hematopoietic stem cells. The first solid evidence for the existence of stem cells, and in particular hematopoietic stem cells, was reported from studies done in mice by Ernest McCulloch and James Till in 1963. In recent years, interest in stem cells has grown enormously, and they are now of interest to almost every area of medicine and the health sciences. A stem cell is a cell with a unique capacity to produce unaltered daughter cells (ie, self-renewal) and to generate specialized cell types (potency). Stem cells may be totipotent (capable of producing all the cells in an organism), pluripotent (able to differentiate into cells of any of the three germ layers), multipotent (produce only cells of a closely related family) or unipotent (produce only one type of cell). Stem cells are also classified as embryonic and adult; the latter are more limited in their capabilities to differentiate than the former, although genetic approaches to overcoming this restriction are becoming available.
FIGURE 52–1 Simplified scheme of differentiation of red blood cells and other blood cells from the hematopoietic stem cell. Sites of action of interleukins (IL-7, IL-3, and IL-5), stem cell factor (SCF), thrombopoietin (TPO), FLT-3 ligand (a growth factor), granulocyte-macrophage colony-stimulating factor (GM-CSF), erythropoietin (EPO), monocyte colony-stimulating factor (M-CSF), and granulocyte colony-stimulating factor (G-CSF) are shown. Sites of action of important transcription factors are not shown. Various steps in the development of lymphoid cells (top part of the figure) have been omitted and abbreviated to one step. (Modified, with permission, from Scadden DT, Longo DL in Fauci AS et al (editors), Harrison’s Principles of Internal Medicine, 17th ed. McGraw-Hill, 2008. Chapter 68)
As shown in Figure 52–1, red blood cells and platelets share a common pathway of differentiation until the stage of megakaryocyte erythroid progenitors. Cells of lymphoid origin branch off at the stage of multipotent progenitors, and other white blood cells at the stage of the common myeloid progenitors. Each pathway is regulated by various factors (eg, stem cell factor, thrombopoietin, various interleukins, erythropoietin, etc), and key specific transcription factors (not indicated in the figure) are also involved at the stages indicated.
Stem cell factor is a cytokine that plays an important role in the proliferation of hematopoietic stem cells and some of their progeny. Thrombopoietin is a glycoprotein that is important in regulating the production of platelets by the bone marrow. Interleukins are cytokines produced by leukocytes; they regulate various aspects of hematopoiesis and of the immune system.
THE RED BLOOD CELL IS SIMPLE IN TERMS OF ITS STRUCTURE & FUNCTION
The major functions of the red blood cell are relatively simple, consisting of delivering oxygen to the tissues and of helping in the disposal of carbon dioxide and protons formed by tissue metabolism. Thus, it has a much simpler structure than most human cells, being essentially composed of a membrane surrounding a solution of hemoglobin (this protein forms about 95% of the intracellular protein of the red cell). There are no intracellular organelles, such as mitochondria, lysosomes, or Golgi apparatus. Human red blood cells, like most red cells of animals, are nonnucleated. However, the red cell is not metabolically inert. ATP is synthesized from glycolysis and is important in processes that help the red blood cell maintain its biconcave shape and also in the regulation of the transport of ions (eg, by the Na+-K+– ATPase and the anion exchange protein [see below]) and of water in and out of the cell. The biconcave shape increases the surface-to-volume ratio of the red blood cell, thus facilitating gas exchange. The red cell contains cytoskeletal components (see below) that play an important role in determining its shape.
About Two Million Red Blood Cells Enter the Circulation per Second
The lifespan of the normal red blood cell is 120 days; this means that slightly less than 1% of the population of red cells (~200 billion cells) is replaced daily (or ~2 million per second). The new red cells that appear in the circulation still contain ribosomes and elements of the endoplasmic reticulum. The RNA of the ribosomes can be detected by suitable stains (such as cresyl blue), and cells containing it are termed reticulocytes; they normally number about 1% of the total red blood cell count. The lifespan of the red blood cell can be dramatically shortened in a variety of hemolytic anemias. The number of reticulocytes is markedly increased in these conditions, as the bone marrow attempts to compensate for rapid breakdown of red blood cells by increasing the amount of new, young red cells in the circulation.
Erythropoietin Regulates Production of Red Blood Cells
Human erythropoietin (EPO) is a glycoprotein of 166 amino acids (molecular mass about 34 kDa). Its amount in plasma can be measured by radioimmunoassay. It is the major regulator of human erythropoiesis (Figure 52–1). As shown in the figure, earlier stages in the development of red blood cells involve stem cell factor, thrombopoietin, and interleukin-3. EPO is synthesized mainly by the kidney and is released in response to hypoxia into the bloodstream, in which it travels to the bone marrow. There it interacts with progenitors of red blood cells via a specific receptor. The receptor is a transmembrane protein consisting of two different subunits and a number of domains. It is not a tyrosine kinase, but it stimulates the activities of specific members of this class of enzymes involved in downstream signal transduction.
The availability of a cDNA for EPO has made it possible to produce substantial amounts of this hormone for analysis and for therapeutic purposes; previously the isolation of erythropoietin from human urine yielded very small amounts of the protein. The major use of recombinant EPO has been in the treatment of a small number of anemic states, such as that due to renal failure. As described in Chapter 47, attempts have been made to prolong the half-life of EPO (thus lengthening its activity) in the circulation by altering the nature of its sugar chains.
MANY GROWTH FACTORS REGULATE PRODUCTION OF WHITE BLOOD CELLS
A large number of hematopoietic growth factors have been identified in recent years in addition to erythropoietin. This area of study adds to knowledge about the differentiation of blood cells, provides factors that may be useful in treatment, and also has implications for understanding of the abnormal growth of blood cells (eg, the leukemias). Like erythropoietin, most of the growth factors isolated have been glycoproteins, are very active in vivo, and in vitro interact with their target cells via specific cell surface receptors, and ultimately (via intracellular signals) affect gene expression, thereby promoting differentiation. Many have been cloned, permitting their production in relatively large amounts. Two of particular interest are granulocyte- and granulocyte-macrophage colony-stimulating factors (G-CSF and GM-CSF, respectively). As indicated in Figure 52–1, G-CSF is relatively specific, inducing mainly granulocytes, whereas GMCSF induces a wider variety of white blood cells. When the production of neutrophils is severely depressed, this condition is referred to as neutropenia. It is particularly likely to occur in patients treated with certain chemotherapeutic regimens and after bone marrow transplantation. These patients are liable to develop overwhelming infections. G-CSF has been administered to such patients to boost production of neutrophils.
THE RED BLOOD CELL HAS A UNIQUE & RELATIVELY SIMPLE METABOLISM
Various aspects of the metabolism of the red cell, many of which are discussed in other chapters of this text, are summarized in Table 52-3.
TABLE 52–3 Summary of Important Aspects of the Metabolism of the Red Blood Cell
The Red Blood Cell Has a Glucose Transporter in Its Membrane
The entry rate of glucose into red blood cells is far greater than would be calculated for simple diffusion. Rather, it is an example of facilitated diffusion (Chapter 40). The specific protein involved in this process is called the glucose transporter (GLUT1) or glucose permease. Some of its properties are summarized in Table 52-4. The process of entry of glucose into red blood cells is of major importance because it is the major fuel supply for these cells. About 12 different but related glucose transporters have been isolated from various human tissues; unlike the red cell transporter, some of these are insulin-dependent (eg, in muscle and adipose tissue). There is considerable interest in the latter types of transporter because defects in their recruitment from intracellular sites to the surface of skeletal muscle cells may help explain the insulin resistance displayed by patients with type 2 diabetes mellitus.
TABLE 52–4 Some Properties of the Glucose Transporter of the Membrane of the Red Blood Cell (GLUT1)
Reticulocytes Are Active in Protein Synthesis
The mature red blood cell cannot synthesize protein. Reticulocytes are active in protein synthesis. Once reticulocytes enter the circulation, they lose their intracellular organelles (ribosomes, mitochondria, etc) within about 24 h, becoming young red blood cells and concomitantly losing their ability to synthesize protein. Extracts of rabbit reticulocytes (obtained by injecting rabbits with a chemical—phenylhydrazine—that causes a severe hemolytic anemia, so that the red cells are almost completely replaced by reticulocytes) are widely used as an in vitro system for synthesizing proteins. Endogenous mRNAs present in these reticulocytes are destroyed by use of a nuclease, whose activity can be inhibited by the addition of Ca2+. The system is then programmed by adding purified mRNAs or whole-cell extracts of mRNAs, and radioactive proteins are synthesized in the presence of 35S-labeled L-methionine or other radiolabeled amino acids. The radioactive proteins synthesized are separated by sodium dodecyl sulfate polyacrylamide gel electrophoresis (SDS-PAGE) and detected by radioautography.
With regard to protein synthesis, it is of interest to note that certain disorders due to genetic abnormalities cause impairment of ribosome structure and function and have been named ribosomopathies. These include some cases of Diamond-Blackfan anemia, in which mutations in a ribosomal RNA processing gene (RPS19) result in red cell hypoplasia. The 5q-syndrome presents with a similar clinical picture and is due to an insufficiency of ribosomal protein RPS 14.
Superoxide Dismutase, Catalase, & Glutathione Protect Blood Cells from Oxidative Stress & Damage
Several powerful oxidants are produced during the course of metabolism, in both blood cells and most other cells of the body. These include superoxide (, hydrogen peroxide (H2O2), peroxyl radicals (ROO•), and hydroxyl radicals (OH•) and are referred to as reactive oxygen species (ROS). Free radicals are atoms or groups of atoms that have an unpaired electron (see Chapters 15 & 45). OH• is a particularly reactive molecule and can react with proteins, nucleic acids, lipids, and other molecules to alter their structure and produce tissue damage. The reactions listed in Table 52-5 play an important role in forming these oxidants and in disposing of them; each of these reactions will now be considered in turn.
TABLE 52–5 Reactions of Importance in Relation to Oxidative Stress in Blood Cells and Various Tissues
Superoxide is formed (reaction 1) in the red blood cell by the auto-oxidation of hemoglobin to methemoglobin (approximately 3% of hemoglobin in human red blood cells has been calculated to auto-oxidize per day); in other tissues, it is formed by the action of enzymes such as cytochrome P450 reductase and xanthine oxidase. When stimulated by contact with bacteria, neutrophils exhibit a respiratory burst (see below) and produce superoxide in a reaction catalyzed by NADPH oxidase (reaction 2). Superoxide spontaneously dismutates to form H2O2 and O2; however, the rate of the same reaction is speeded up tremendously by the action of the enzyme superoxide dismutase (reaction 3). Hydrogen peroxide is subject to a number of fates. The enzyme catalase, present in many types of cells, converts it to H2O and O2 (reaction 4). Neutrophils possess a unique enzyme, myeloperoxidase, which uses H2O2 and halides to produce hypohalous acids (reaction 5); this subject is discussed further below. The selenium-containing enzyme glutathione peroxidase (Chapter 21) will also act on reduced glutathione (GSH) and H2O2 to produce oxidized glutathione (GSSG) and H2O (reaction 6); this enzyme can also use other peroxides as substrates. OH• and OH– can be formed from H2O2 in a nonenzymatic reaction catalyzed by Fe2+ (the Fenton reaction, reaction 7). and H2O2 are the substrates in the iron-catalyzed Haber-Weiss reaction (reaction 8), which also produces OH• and OH–. Superoxide can release iron ions from ferritin. Thus, production of OH. may be one of the mechanisms involved in tissue injury due to iron overload in hemochromatosis (see Case no. 10 Chapter 57).
Chemical compounds and reactions capable of generating potential toxic oxygen species can be referred to as prooxidants. On the other hand, compounds and reactions disposing of these species, scavenging them, suppressing their formation, or opposing their actions are antioxidants and include compounds such as NADPH, GSH, ascorbic acid, and vitamin E. In a normal cell, there is an appropriate prooxidant:antioxidant balance. However, this balance can be shifted toward the pro-oxidants when production of oxygen species is increased greatly (eg, following ingestion of certain chemicals or drugs) or when levels of antioxidants are diminished (eg, by inactivation of enzymes involved in disposal of oxygen species and by conditions that cause low levels of the antioxidants mentioned above). This state is called “oxidative stress” (see Chapter 45) and can result in serious cell damage if the stress is massive or prolonged.
ROS are now thought to play an important role in many types of cellular injury (eg, resulting from administration of various toxic chemicals or from ischemia), some of which can result in cell death. Indirect evidence supporting a role for these species in generating cell injury is provided if administration of an enzyme such as superoxide dismutase or catalase is found to protect against cell injury in the situation under study.
Deficiency of Glucose-6-Phosphate Dehydrogenase Is Frequent in Certain Areas & Is an Important Cause of Hemolytic Anemia
NADPH, produced in the reaction catalyzed by the X-linked glucose-6-phosphate dehydrogenase (Table 52-5, reaction 9) in the pentose phosphate pathway (Chapter 21), plays a key role in supplying reducing equivalents in the red cell and in other cells such as the hepatocyte. Since the pentose phosphate pathway is virtually its sole means of producing NADPH, the red blood cell is very sensitive to oxidative damage if the function of this pathway is impaired (eg, by enzyme deficiency). One function of NADPH is to reduce GSSG to GSH, a reaction catalyzed by glutathione reductase (reaction 10).
Deficiency of the activity of glucose-6-phosphate dehydrogenase, owing to mutation, is extremely frequent in some regions of the world (eg, tropical Africa, the Mediterranean, certain parts of Asia, and in North America among blacks). It is the most common of all enzymopathies (diseases caused by abnormalities of enzymes), and some 140 genetic variants of the enzyme have been distinguished; at least 400 million people are estimated to have a variant gene. It is thought that an abnormal form of this enzyme confers resistance to malaria. The disorder resulting from deficiency of glucose-6-phosphate dehydrogenase is hemolytic anemia. When an abnormal form of an enzyme causes pathology it is referred to as an enzymopathy. Consumption of broad beans (Vicia faba) by individuals deficient in activity of the enzyme can precipitate an acute attack of hemolytic anemia because they contain potential oxidants. In addition, a number of drugs (eg, the antimalarial drug primaquine [the condition caused by intake of primaquine is called primaquine-sensitive hemolytic anemia] and sulfonamides) and chemicals (eg, naphthalene) precipitate an attack, because their intake leads to generation of H2O2 or Normally, H2O2 is disposed of by catalase and glutathione peroxidase (Table 52-5, reactions 4 and 6), the latter causing increased production of GSSG. GSH is regenerated from GSSG by the action of the enzyme glutathione reductase, which depends on the availability of NADPH (reaction 10). The red blood cells of individuals who are deficient in the activity of glucose-6-phosphate dehydrogenase cannot generate sufficient NADPH to regenerate GSH from GSSG, which in turn impairs their ability to dispose of H2O2 and of oxygen radicals. These compounds can cause oxidation of critical SH groups in proteins and possibly peroxidation of lipids in the membrane of the red cell, causing lysis of the red cell membrane. Some of the SH groups of hemoglobin become oxidized, and the protein precipitates inside the red blood cell, forming Heinz bodies
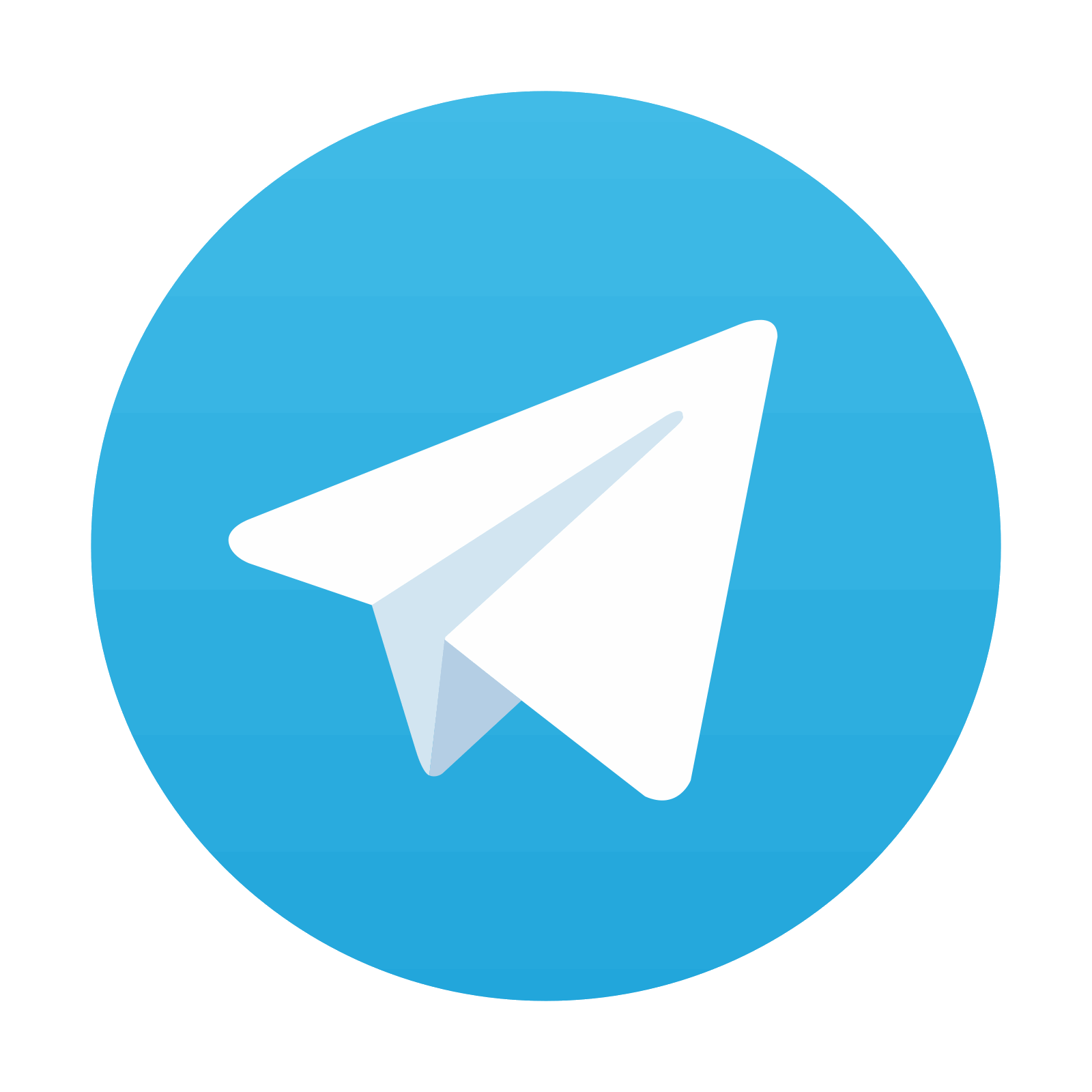
Stay updated, free articles. Join our Telegram channel
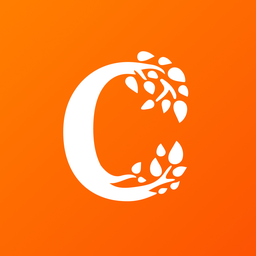
Full access? Get Clinical Tree
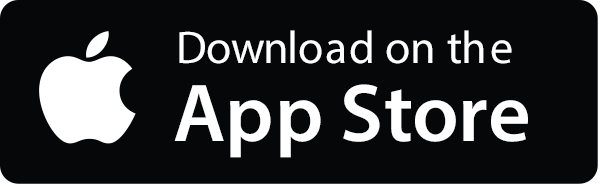
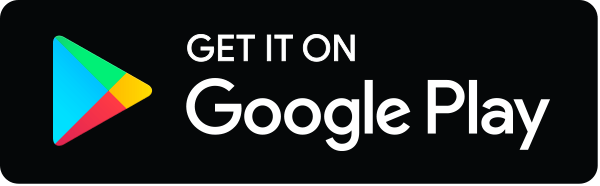