Proteolytic enzymes (also called proteases) break down dietary proteins into their constituent amino acids in the stomach and the intestine. Many of these digestive proteases are synthesized as larger, inactive forms known as zymogens. After zymogens are secreted into the digestive tract, they are cleaved to produce the active proteases.
In the stomach, pepsin begins the digestion of proteins by hydrolyzing them to smaller polypeptides. The contents of the stomach pass into the small intestine, where enzymes produced by the exocrine pancreas act. The pancreatic proteases (trypsin, chymotrypsin, elastase, and the carboxypeptidases) cleave the polypeptides into oligopeptides and amino acids.
Further cleavage of the oligopeptides to amino acids is accomplished by enzymes produced by the intestinal epithelial cells. These enzymes include aminopeptidases located on the brush border and other peptidases located within the cells. Ultimately, the amino acids produced by protein digestion are absorbed through the intestinal epithelial cells and enter the blood.
A large number of overlapping transport systems exist for amino acids in cells. Some systems contain facilitative transporters, whereas others express sodium-linked transporters, which allow the active transport of amino acids into cells. Defects in amino acid transport can lead to disease.
Proteins are also continually synthesized and degraded (turnover) in cells. A wide variety of proteases in cells carry out this activity. Lysosomal proteases (cathepsins) degrade proteins that enter lysosomes. Cytoplasmic proteins targeted for turnover are covalently linked to the small protein ubiquitin, which then interacts with a large protein complex, the proteasome, to degrade the protein in an adenosine triphosphate (ATP)–dependent process. The amino acids released from proteins during turnover can then be used for the synthesis of new proteins or for energy generation.
THE WAITING ROOM 
Susan F., a young child with cystic fibrosis (see Chapter 16), has had repeated bouts of bronchitis/bronchiolitis caused by Pseudomonas aeruginosa. With each of these infections, her response to aerosolized antibiotics has been good. However, her malabsorption of food continues, resulting in foul-smelling, glistening, bulky stools. Her growth records show a slow decline. She is now in the 24th percentile for height and the 20th percentile for weight. She is often listless and irritable, and she tires easily. When her pediatrician discovered that her levels of the serum proteins albumin and prealbumin were low to low-normal (indicating protein malnutrition), Susan F. was given enteric-coated microspheres of pancreatic enzymes. Almost immediately, the character of Susan F.’s stools became more normal and she began gaining weight. Over the next 6 months, her growth curves showed improvement, and she seemed brighter, more active, and less irritable.
For the first few months after a painful episode of renal colic, during which he passed a kidney stone (see Chapter 6), David K. had faithfully maintained a high daily fluid intake and had taken the medication required to increase the pH of his urine. He had been diagnosed with cystinuria, a genetically determined amino acid substitution in the transport protein that normally reabsorbs cysteine, arginine, and lysine from the kidney lumen back into the renal tubular cells. Therefore, his urine contained high amounts of these amino acids. Cystine, which is less soluble than the other amino acids, precipitates in the urine to form renal stones (also known as calculi). The measures he was instructed to follow were necessary to increase the solubility of the large amounts of cystine present in his urine and thereby to prevent further formation of kidney stones. With time, however, it became difficult for him to keep up with his preventive program. After failing to take his medication for a month, he experienced another severe episode of renal colic with grossly bloody urine. Fortunately, he passed the stone spontaneously, after which he tried again to adhere to his treatment regimen.
His mother heard that some dietary amino acids were not absorbed in patients with cystinuria and asked whether any dietary changes would reduce David K.’s chances of developing additional renal stones.
I. Protein Digestion
The digestion of proteins begins in the stomach and is completed in the intestine (Fig. 35.1). The enzymes that digest proteins are produced as inactive precursors (zymogens) that are larger than the active enzymes. The inactive zymogens are secreted from the cells in which they are synthesized and enter the lumen of the digestive tract, where they are cleaved to smaller forms that have proteolytic activity (Fig. 35.2). These active enzymes have different specificities; no single enzyme can completely digest a protein. However, by acting in concert, they can digest dietary proteins to amino acids and small peptides, which are cleaved by peptidases associated with intestinal epithelial cells.
FIGURE 35.1 Digestion of proteins. The proteolytic enzymes, pepsin, trypsin, chymotrypsin, elastase, and the carboxypeptidases, are produced as zymogens (the “pro” and “ogen,” in red, accompanying the enzyme name) that are activated by cleavage after they enter the gastrointestinal lumen (see Fig. 35.2). Pepsinogen is produced within the stomach and is activated within the stomach (to pepsin) as the pH drops due to HCl secretion. HCl, hydrochloric acid.
FIGURE 35.2 Activation of the gastric and pancreatic zymogens. Pepsinogen catalyzes its own cleavage as the pH of the stomach drops. Trypsinogen is cleaved by enteropeptidase in the intestine to form the active protease trypsin. Trypsin then plays a key role by catalyzing the cleavage and activation of the other pancreatic zymogens.
A. Digestion in the Stomach
Pepsinogen is secreted by the chief cells of the stomach. The gastric parietal cells secrete hydrochloric acid (HCl). The acid in the stomach lumen alters the conformation of pepsinogen so that it can cleave itself, producing the active protease pepsin. Thus, the activation of pepsinogen is autocatalytic.
Dietary proteins are denatured by the acid in the stomach. This inactivates the proteins and partially unfolds them so that they are better substrates for proteases. However, at the low pH of the stomach, pepsin is not denatured and acts as an endopeptidase, cleaving peptide bonds at various points within the protein chain. Although pepsin has fairly broad specificity, it tends to cleave peptide bonds in which the carboxyl group is provided by an aromatic or acidic amino acid (Fig. 35.3). Smaller peptides and some free amino acids are produced.
FIGURE 35.3 Action of the digestive proteases. Pepsin, trypsin, chymotrypsin, and elastase are endopeptidases; they hydrolyze peptide bonds within chains. The others are exopeptidases; aminopeptidases remove the amino acid at the N terminus, and the carboxypeptidases remove the amino acid at the C-terminus. For each proteolytic enzyme, the amino acid residues involved in the peptide bond that is cleaved are listed beside the R group to the right of the enzyme name.
B. Digestion by Enzymes from the Pancreas
As the gastric contents empty into the intestine, they encounter the secretions from the exocrine pancreas. Recall that the exocrine pancreas secretes amylase for starch digestion and lipase and colipase for dietary triacylglycerol digestion. Another pancreatic secretion is bicarbonate, which, in addition to neutralizing stomach acid, raises the pH so that the pancreatic proteases, which are also present in pancreatic secretions, can be active. As secreted, these pancreatic proteases are in the inactive proenzyme form (zymogens). Because the active forms of these enzymes can digest each other, it is important for their zymogen forms all to be activated within a short span of time. This is accomplished by the cleavage of trypsinogen to the active enzyme trypsin, which then cleaves the other pancreatic zymogens, producing their active forms (see Fig. 35.2).
The zymogen trypsinogen is cleaved to form trypsin by enteropeptidase (a protease, formerly called enterokinase) secreted by the brush border cells of the small intestine. Trypsin catalyzes the cleavages that convert chymotrypsinogen to the active enzyme chymotrypsin, proelastase to elastase, and the procarboxypeptidases to the carboxypeptidases. Thus, trypsin plays a central role in digestion because it both cleaves dietary proteins and activates other digestive proteases produced by the pancreas.
Trypsin, chymotrypsin, and elastase are serine proteases (see Chapter 9) that act as endopeptidases. Trypsin is the most specific of these enzymes, cleaving peptide bonds in which the carboxyl (carbonyl) group is provided by lysine or arginine (see Fig. 35.3). Chymotrypsin is less specific but favors residues that contain hydrophobic amino acids. Elastase cleaves not only elastin (for which it was named) but also other proteins at bonds in which the carboxyl group is contributed by amino acid residues with small side chains (alanine, glycine, and serine). The actions of these pancreatic endopeptidases continue the digestion of dietary proteins begun by pepsin in the stomach.
The smaller peptides formed by the action of trypsin, chymotrypsin, and elastase are attacked by exopeptidases, which are proteases that cleave one amino acid at a time from the end of the chain. Procarboxypeptidases, zymogens produced by the pancreas, are converted by trypsin to the active carboxypeptidases. These exopeptidases remove amino acids from the carboxyl ends of peptide chains. Carboxypeptidase A preferentially releases hydrophobic amino acids, whereas carboxypeptidase B releases basic amino acids (arginine and lysine).
C. Digestion by Enzymes from Intestinal Cells
Exopeptidases produced by intestinal epithelial cells act within the brush border and also within the cell. Aminopeptidases, located on the brush border, cleave one amino acid at a time from the amino end of peptides. Intracellular peptidases act on small peptides that are absorbed by the cells.
The concerted action of the proteolytic enzymes produced by cells of the stomach, pancreas, and intestine cleaves dietary proteins to amino acids. The digestive enzymes digest themselves as well as dietary protein. They also digest the intestinal cells that are regularly sloughed off into the lumen. These cells are replaced by cells that mature from precursor cells in the duodenal crypts. The amount of protein that is digested and absorbed each day from digestive juices and cells released into the intestinal lumen may be equal to, or greater than, the amount of protein consumed in the diet (50 to 100 g).
II. Absorption of Amino Acids
Amino acids are absorbed from the intestinal lumen through secondary active Na+-dependent transport systems and through facilitated diffusion.
A. Cotransport of Sodium Ions and Amino Acids
Amino acids are absorbed from the lumen of the small intestine principally by semispecific Na+-dependent transport proteins in the luminal membrane of the intestinal cell brush border, similar to that already seen for carbohydrate transport (Fig. 35.4). The cotransport of Na+ and the amino acid from the outside of the apical membrane to the inside of the cell is driven by the low intracellular Na+ concentration. Low intracellular Na+ concentration results from the pumping of Na+ out of the cell by a Na+, K+-ATPase on the serosal membrane. Thus, the primary transport mechanism is the creation of a sodium gradient; the secondary transport process is the coupling of amino acids to the influx of sodium. This mechanism allows the cells to concentrate amino acids from the intestinal lumen. The amino acids are then transported out of the cell into the interstitial fluid principally by facilitated transporters in the serosal membrane (see Fig. 35.4).
FIGURE 35.4 Transepithelial amino acid transport. Na+-dependent carriers transport both Na+ and an amino acid into the intestinal epithelial cell from the intestinal lumen. Na+ is pumped out on the serosal side (across the basolateral membrane) in exchange for K+ by the Na+, K+-ATPase. On the serosal side, the amino acid is carried by a facilitated transporter down its concentration gradient into the blood. This process is an example of secondary active transport. ADP, adenosine diphosphate; ATP, adenosine triphosphate; Pi, inorganic phosphate.
At least six different Na+-dependent amino acid carriers are located in the apical brush border membrane of the epithelial cells. These carriers have an overlapping specificity for different amino acids. One carrier preferentially transports neutral amino acids, another transports proline and hydroxyproline, a third preferentially transports acidic amino acids, and a fourth transports basic amino acids (lysine, arginine, and the urea cycle intermediate ornithine) and cystine (two cysteine residues linked by a disulfide bond). In addition to these Na+-dependent carriers, some amino acids are transported across the luminal membrane by facilitated transport carriers. Most amino acids are transported by more than one transport system.
As with glucose transport, the Na+-dependent carriers of the apical membrane of the intestinal epithelial cells are also present in the renal epithelium. However, different isozymes are present in the cell membranes of other tissues. Conversely, the facilitated transport carriers in the serosal membrane of the intestinal epithelia are similar to those found in other cell types in the body. During starvation, the intestinal epithelia, like these other cells, take up amino acids from the blood to use as an energy source. Thus, amino acid transport across the serosal membrane is bidirectional.
B. Transport of Amino Acids into Cells
Amino acids that enter the blood are transported across cell membranes of the various tissues principally by Na+-dependent cotransporters and, to a lesser extent, by facilitated transporters (Table 35.1). In this respect, amino acid transport differs from glucose transport, which is Na+-dependent transport in the intestinal and renal epithelium but facilitated transport in other cell types. The Na+ dependence of amino acid transport in liver, muscle, and other tissues allows these cells to concentrate amino acids from the blood. These transport proteins have a different genetic basis, amino acid composition, and somewhat different specificity than those in the luminal membrane of intestinal epithelia. They also differ somewhat among tissues. For instance, the N-system for glutamine uptake is present in the liver, but either is not present in other tissues or is present as an isoform with different properties. As with the epithelial cell transporters, there is also some overlap in specificity of the transport proteins, with most amino acids being transported by more than one carrier.
TABLE 35.1 A Partial Listing of Amino Acid Transport Systemsa
SYSTEM NAME | SODIUM-DEPENDENT? | SPECIFICITY | TISSUES EXPRESSED |
A | Yes | Small and polar neutral amino acids (Ala, Ser, Gln, Gly, Pro, Cys, Asn, His, Met) | Many |
ASC | Yes | Small amino acids (Ala, Ser, Cys) | Many |
N | Yes | Gln, Asn, His | Liver, basolateral membrane of kidney |
L | No | Branched and aromatic amino acids (His, Met, Leu, Ile, Val, Phe, Tyr, Trp) | Manyb |
B0,+ | Yes | Basic amino acids | Intestine (brush border)c and kidney |
B0 | Yes | Zwitterionic amino acids (monoamino, monocarboxylic acid amino acids) | Intestine and kidneyd |
XAG− | Yes | Anionic amino acids (Asp, Glu) | Intestine (brush border) and kidney |
Imino | Yes | Pro, hydroxyproline, Gly | Intestine (brush border) and kidney |
aNot all transport systems are listed.
bThis transport system will be exploited in a treatment for phenylketonuria (PKU); see Chapter 37.
cThis system is most likely defective in cystinuria.
dThis system is most likely defective in Hartnup disease.
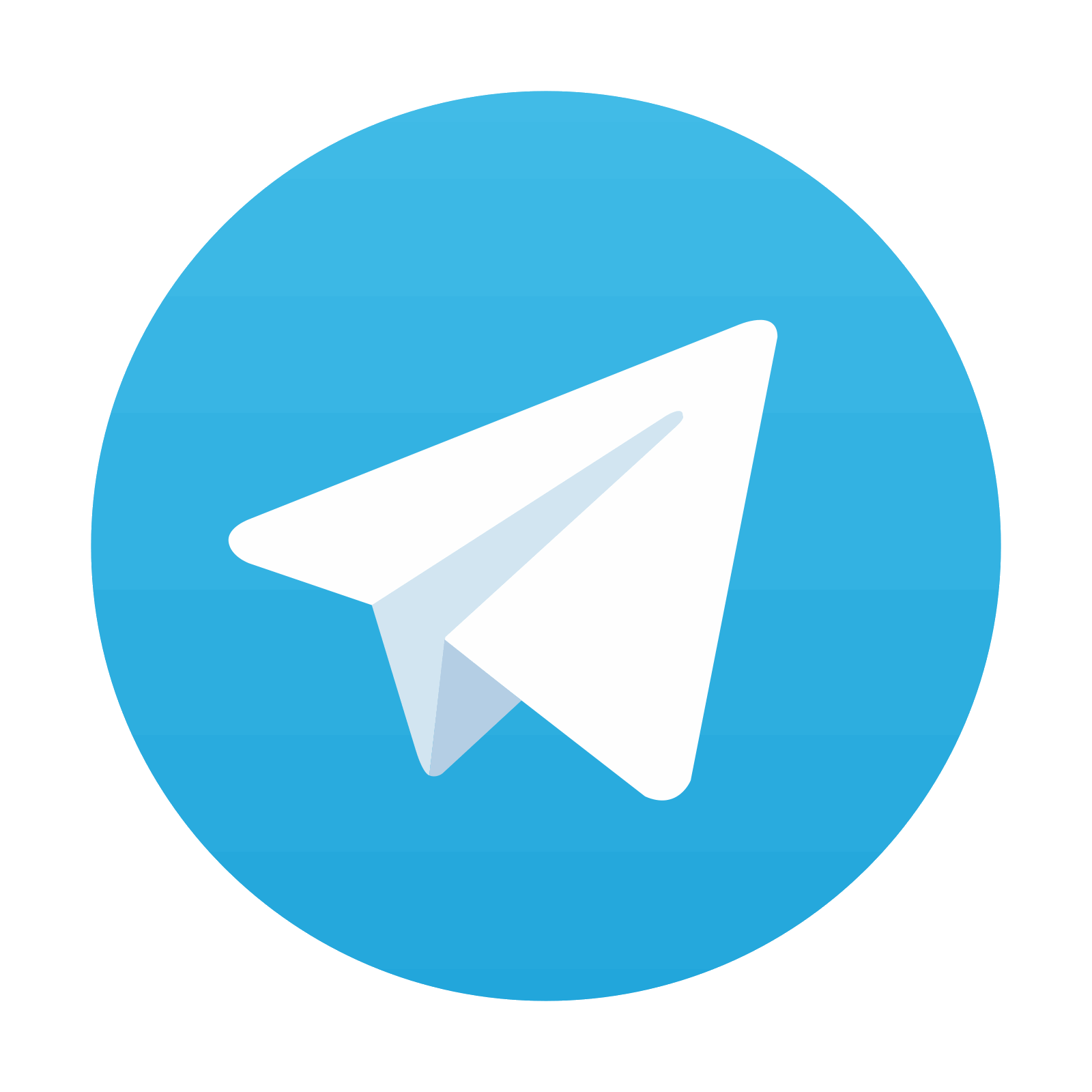
Stay updated, free articles. Join our Telegram channel
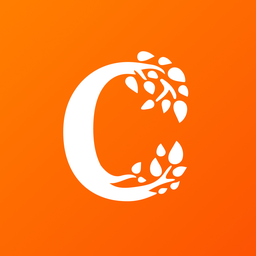
Full access? Get Clinical Tree
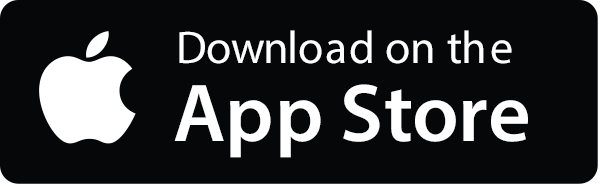
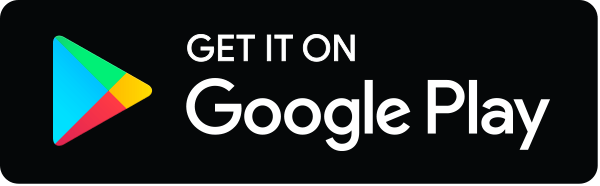