Figure 2-1. The chemical reactions of glycogenesis and glycogenolysis. Glucose-6-phosphatase allows hepatic glucose to be transported out of the hepatocyte for use in other tissues. Glucose-6-phosphate, in red, plays a central role in carbohydrate metabolism.
Figure 2-2. Glucagon-stimulated enzyme cascade, responsible for the control of glycogen metabolism. Inactive forms are shown in black, active forms in blue.
The aerobic conversion of glucose to pyruvate has three effects: (a) a net gain of two ATP molecules, (b) generation of two reducing equivalents of the nicotinamide adenine nucleotide (NADH + H+), and usually, (c) conversion of pyruvate to acetyl CoA with subsequent conversion of acetyl CoA in the mitochondria to ATP. The conversion of glucose to pyruvate is regulated by three enzymes: hexokinase (glucokinase), phosphofructokinase, and pyruvate kinase, which are nonequilibrium reactions and as such, functionally irreversible.
Under anaerobic conditions, NADH cannot be reoxidized by transfer of reducing equivalents through the electron transport chain to oxygen. Instead, pyruvate is reduced by NADH to lactate. Glycolysis takes place in the cytoplasm, in contrast to the citric acid cycle and oxidative phosphorylation which are mitochondrial processes. During times of glucose excess, as in the fed state, hepatic glycolysis can generate energy in the form of ATP, but the oxidation of ketoacids is a preferred energy source in liver.
The conversion of lactate (through pyruvate) to glucose – a process possible only in the presence of oxygen – is an important means of preventing severe lactic acidosis. Active skeletal muscles and erythrocytes form large quantities of lactate. In patients with large wounds, lactate also accumulates. The liver is exceptionally efficient at converting lactate to pyruvate through the Cori cycle (Fig. 2-4). As a result, one would expect that only significant liver dysfunction would affect the Cori cycle and lead to hyperlactatemia. However, lactate levels are now widely used to assess shock – septic and otherwise.2 The hypothesis is that circulatory hypoperfusion impairs tissue oxygen delivery with resultant mitochondrial hypoxia. In the absence of adequate oxygen, mitochondria switch to anaerobic glycolysis and oxidative phosphorylation stops. As a result, serum lactate concentrations appear proportional to ongoing tissue oxygenation deficits; thus improved lactate clearance can be used as a surrogate for success of sepsis therapy.3 Serum lactate can also be used to assess prognosis and triage patients to ICU level care.4
Figure 2-3. The glycolytic pathway. There is a net gain of two ATP molecules per glucose molecule. Phosphofructokinase is the key regulatory enzyme in this pathway; however, all the enzymes in red catalyze irreversible reactions. The pathway shown here is active only in the presence of aerobic conditions.
In erythrocytes, a unique variant of glycolysis enhances oxyhemoglobin dissociation. The first site in glycolysis for generation of ATP is bypassed, leading to the formation of 2,3-bisphosphoglycerate by an additional enzyme called bisphosphoglycerate mutase. Kinetics of the mutase present in erythrocytes allow the presence of high concentrations of 2,3-bisphosphoglycerate to build up. The 2,3-bisphosphoglycerate displaces oxygen from hemoglobin, allowing a shift of the oxyhemoglobin dissociation curve to the right.
Gluconeogenesis
There is an absolute minimum requirement for glucose in humans. Below a certain blood glucose concentration, brain dysfunction causes coma and death. When glucose becomes scarce, as in the fasting state, glycogenolysis occurs. Once glycogen stores have been depleted, the liver and kidneys are capable of synthesizing new glucose by the process of gluconeogenesis. Glucagon is produced in response to low blood sugar levels and stimulates gluconeogenesis.
Figure 2-4. The gluconeogenesis pathway. The irreversible nature of the glycolytic pathway means that a different sequence of biosyntheses is required for glucose production. The enzymes in red catalyze irreversible reactions that are different from those in glycolysis. In mammals, glucose cannot be synthesized from acetyl coenzyme A, only from cytosolic pyruvate.
Gluconeogenesis is not a simple reversal of the glycolytic pathway. In glycolysis, as noted previously, the conversion of glucose to pyruvate is a one-way reaction. As a result, four separate, functionally irreversible enzyme reactions are required to convert pyruvate into glucose (Fig. 2-5). These enzymes are pyruvate carboxylase, phosphoenolpyruvate carboxykinase, fructose-1,6-bisphosphatase, and glucose-6-phosphatase. Other enzymes are shared with the glycolytic pathway.
About 60% of the naturally occurring amino acids, glycerol, or lactate can also be used as substrates for glucose production. Alanine is the amino acid most easily converted into glucose. Simple deamination allows conversion to pyruvate, which is subsequently converted to glucose. Other amino acids can be converted into three-, four-, or five-carbon sugars and then enter the phosphogluconate pathway (next section). Gluconeogenesis is enhanced by fasting, critical illness, and periods of anaerobic metabolism.
Figure 2-5. The Cori cycle, an elegant mechanism for the hepatic conversion of muscle lactate into new glucose. Pyruvate plays a key role in this process.
Phosphogluconate Pathway
When glucose enters the liver, glycogen is formed until the hepatic glycogen capacity is reached (about 100 g). If excess glucose is still available, the liver converts it to fat by the phosphogluconate pathway (also known as the pentose phosphate pathway) (Fig. 2-6). The cytosolic phosphogluconate pathway can completely oxidize glucose, generating CO2 and nicotinamide adenine dinucleotide phosphate (NADPH) through what is known as the oxidative phase. Hydrogen atoms released in the phosphogluconate pathway combine with oxidized nicotinamide adenine dinucleotide phosphate (NADP+) to form reduced nicotinamide adenine dinucleotide phosphate (NADPH − H+).5 The oxidative phase is present only in tissues, such as the adrenal glands and gonads, that require reductive biosyntheses such as steroidogenesis or other forms of lipid synthesis. Essentially, all tissues contain the nonoxidative phase, which is reversible and produces ribose precursors for nucleotide synthesis. In erythrocytes, the phosphogluconate pathway provides reducing equivalents for the production of reduced glutathione by glutathione reductase. Reduced glutathione can remove hydrogen peroxide, which increases the conversion of oxyhemoglobin to methemoglobin and subsequent hemolysis.
LIPID METABOLISM
Lipid Transport
Lipid transport throughout the body is made complicated by the fact that lipids are insoluble in water. To overcome this physicochemical incompatibility, dietary triglycerides are first split into monoglycerides and fatty acids by the action of intestinal lipases. After absorption into small intestinal cells, triacylglycerols are reformed and aggregate into chylomicrons, which then enter the bloodstream by way of lymph. Chylomicrons are removed from the blood by the liver and adipose tissue. The capillary surface of the liver contains large amounts of lipoprotein lipase, which hydrolyzes triglycerides into fatty acids and glycerol. The fatty acids freely diffuse into hepatocytes for further metabolism. Similar to chylomicrons, very low-density lipoproteins (VLDLs) are synthesized by the liver and are the main vehicle for transport of triacylglycerols to extrahepatic tissues. The intestines and liver are the only two tissues capable of secreting lipid particles. In addition to chylomicrons and VLDLs, there are two other major groups of plasma lipoproteins: low-density lipoproteins (LDLs) and high-density lipoproteins (HDLs). LDLs and HDLs contain predominantly cholesterol and phospholipid.
Figure 2-6. The phosphogluconate pathway. One of the major purposes of this pathway is to generate reduced nicotinamide adenine dinucleotide, which can serve as an electron donor and allow the liver to perform reductive biosynthesis. Glucose-6-phosphate, in red, plays a central role in carbohydrate metabolism.
The structure of all classes of lipoproteins is similar. There is a core of nonpolar lipids, either triacylglycerols or cholesteryl esters, depending on the particular lipoprotein. This nonpolar core is coated with a surface layer of amphipathic phospholipid or cholesterol oriented so that the polar ends are in contact with the plasma. A protein component is also present. The A apolipoproteins occur in chylomicrons and HDLs. The B apolipoproteins come in two forms: B-100 is the predominant apolipoprotein of LDLs, whereas the shorter B-48 is located in chylomicrons. The C apolipoproteins can transfer between VLDLs, LDLs, and HDLs. Apolipoproteins D and E also exist. Apolipoproteins have several functions in lipid transport and storage. Some, such as the B apolipoproteins, are an integral part of the lipoprotein structure. Other apolipoproteins are enzyme cofactors, such as C-II for lipoprotein lipase. Lastly, the apolipoproteins act as ligands for cell surface receptors. As an example, both B-100 and E serve as ligands for the LDL receptor.6
Plasma variations in LDL cholesterol, HDL cholesterol, and triglycerides affect risk for atherosclerotic cardiovascular disease. As dyslipidemias are being identified and studied, new therapeutic approaches are needed. A convergence of human genetics and functional biology has led to recent advances in the study of lipoprotein metabolism. Genome-wide association studies have identified about 100 genes associated with plasma lipid phenotypes – many of which were not previously known to be associated with lipids. These genes are now being functionally validated through human genetic analysis such as deep targeted resequencing of kindreds with Mendelian lipid abnormalities or gene manipulation (over- or underexpression) in cultured cells and animal models.7
FATTY ACID METABOLISM
Most human fatty acids in plasma are long-chain acids (C-16 to C-20). Because long-chain fatty acids are not readily absorbed by the intestinal mucosa, they must first be incorporated into chylomicrons. In contrast, short-chain and medium-chain fatty acids are absorbed directly into the portal circulation and are avidly taken up by the liver. Free fatty acids in the circulation are noncovalently bound to albumin and are transferred to the hepatocyte cytosol by way of fatty acid–binding proteins. Fatty acid-CoA esters are synthesized in the cytosol after hepatic uptake of fatty acids. These fatty acid-CoA esters can be converted into triglyceride, transported into mitochondria for the production of acetyl CoA and reducing equivalents, or stored in the liver as triglycerides. The rate-limiting step in the synthesis of triglyceride is the conversion of acetyl CoA to malonyl CoA. Malonyl CoA, in turn, inhibits the mitochondrial uptake of fatty acid-CoA ester, favoring triglyceride synthesis. The liver also contains dehydrogenases that can unsaturate essential dietary fatty acids. Structural elements of all tissues contain significant amounts of unsaturated fats, and the liver is responsible for the production of these unsaturated fatty acids. As another example, dietary linoleic acid is elongated and dehydrogenated to the prostaglandin precursor arachidonic acid.
Figure 2-7. Diagram of hepatic fatty acid metabolism. Both dietary and newly synthesized fatty acids are esterified and subsequently degraded in the mitochondria for energy, first as reducing equivalents, then adenosine triphosphate via the electron transport chain. Acetyl CoA, in red, plays a central role in lipid metabolism.
3 Free fatty acids are a direct source of energy for cardiac and skeletal muscles and under basal conditions, most free fatty acids are catabolized for energy. Under conditions of adipocyte lipolysis, the liver can take up and metabolize fatty acids. Although fatty acid synthesis occurs in the cytosol, fatty acid oxidation occurs in the mitochondria. Fatty acid-CoA esters bind carnitine, a carrier molecule, and in the absence of cytosolic malonyl CoA, they enter the mitochondria, where they undergo beta-oxidation to acetyl CoA and reducing equivalents (Fig. 2-7). Acetyl CoA can then take one of the following routes: (a) enter the tricarboxylic acid cycle and be degraded to carbon dioxide, (b) be converted to citrate for fatty acid synthesis, or (c) be converted into 3-hydroxy-3-methylglutaryl CoA (HMG-CoA), a precursor of cholesterol and ketone bodies. The mitochondrial hydrolysis of fatty acids is a source of large quantities of ATP. The conversion of stearic acid to carbon dioxide and water, for instance, generates 136 molecules of ATP and demonstrates the highly efficient storage of energy as fat. By a process called beta-oxidation, acetyl-CoA molecules are cleaved from fatty acids. The acetyl CoA is then metabolized through the citric acid cycle under normal circumstances.
In times of significant lipolysis – starvation, uncontrolled diabetes, or other conditions of triglyceride mobilization from adipocyte stores – the predominant ketone bodies 3-hydroxybutyrate and acetoacetate are formed in hepatic mitochondria from free fatty acids and are a source of energy for extrahepatic tissues. Ketogenesis is regulated primarily by the rate of mobilization of free fatty acids. Once in the liver mitochondria, the relative proportion of acyl CoA destined to undergo beta-oxidation is limited by the activity of an enzyme, carnitine palmitoyltransferase-1. Lastly, there are mechanisms that keep the levels of acetyl CoA entering the citric acid cycle constant, so that only at high mitochondrial levels will acetyl CoA be converted to ketone bodies. Even the brain, in times of starvation, can use ketone bodies for half of its energy requirements. At some point, however, the ability of liver to perform beta-oxidation may be inadequate. Under such circumstances, hepatic storage of triglyceride or fatty infiltration of the liver can be significant, leading to the development of nonalcoholic steatohepatitis. Triglyceride storage by itself does not appear to be a cause of hepatic fibrosis, but fatty infiltration may be a marker for the derangement of normal processes by alcohol or drug toxicity, diabetes, or long-term total parenteral nutrition. A specific type of microvesicular fatty accumulation is also seen in a variety of diseases, such as Reye syndrome, morbid obesity, and acute fatty liver of pregnancy.
Figure 2-8. The LDL receptor, an example of a transmembrane receptor that participates in receptor-mediated endocytosis. The LDL receptor specifically binds lipoproteins that contain apolipoprotein B-100 or E. Once internalized, the lipoproteins are degraded. AA, amino acids; EGF, epidermal growth factor.
As noted above, fatty acids are critical elements of all mammalian cells; as energy substrate, in cellular structure, and for intracellular signaling. Evolutionarily, storage of excess fat in adipose tissue mitigated starvation. But in most modern societies the ready availability of calorie-dense foods has led to an epidemic of obesity as is discussed in detail in other chapters. In terms of intermediary metabolism, excess dietary fatty acids are now known to cause insulin resistance in muscle through intramyocellular triglyceride content leading to type II diabetes. This effect is likely due to intracellular perturbations in active lipid metabolites such as diacylglycerols or ceramides. Other studies have documented mitochondrial abnormalities possibly through interference with serine kinases.8
CHOLESTEROL METABOLISM
Cholesterol is an important regulator of membrane fluidity and is a substrate for bile acid and steroid hormone synthesis. Cholesterol may be available by dietary intake or by de novo synthesis. In mammals, mostly new cholesterol is synthesized in the liver from its precursor, acetyl CoA. Dietary cholesterol intake can suppress endogenous synthesis by inhibiting the rate-limiting enzyme in the cholesterol biosynthetic pathway, HMG-CoA reductase. A competitive antagonist, lovastatin, can also block HMG-CoA reductase and effectively lower plasma cholesterol by blocking cholesterol synthesis, stimulating LDL receptor synthesis, and allowing an increased hepatic uptake and metabolism of cholesterol-rich LDL lipoproteins. The structure of the LDL receptor is known and serves as a model for the structure and function of other cell membrane receptors (Fig. 2-8).
Cholesterol is lipophilic and hydrophobic, and most plasma cholesterol is in lipoproteins esterified with oleic or palmitic acid. The liver can process cholesterol esters from all classes of lipoproteins. Hepatocytes can also take up chylomicron remnants containing dietary cholesterol esters. Abnormally elevated levels of cholesterol in VLDLs or LDLs are associated with atherosclerosis, whereas high HDL levels are protective. Newly synthesized hepatic cholesterol is also used to synthesize bile acids for further intestinal absorption of dietary fats. A large proportion of the bile acids secreted by the liver into bile are returned to the liver via the enterohepatic circulation (Fig. 2-9).
Phospholipids
The three major classes of phospholipids synthesized by the liver are lecithins, cephalins, and sphingomyelins. Although most cells in the body are capable of some phospholipid synthesis, the liver produces 90%. Phospholipid formation is controlled by the overall rate of fat metabolism and by the availability of choline and inositol. The main role of phospholipids of all types is to form plasma and organelle membranes. The amphiphilic nature of phospholipids makes them essential for reducing surface tension between membranes and surrounding fluids. Phosphatidylcholine, one of the lecithins, is the major biliary phospholipid and is important in promoting the secretion of free cholesterol into bile. Thromboplastin, one of the cephalins, is needed to initiate the clotting cascade. The sphingomyelins are necessary for the formation of the myelin nerve sheath.
Figure 2-9. The enterohepatic circulation of bile acids. The primary bile acids, cholic acid, and chenodeoxycholic acid, are synthesized in the liver from cholesterol. Deoxycholic acid and lithocholic acid are formed in the colon (blue lines) during bacterial degradation of the primary bile acids. All four bile acids are conjugated with glycine or taurine in the liver. Most of the lithocholic acid is also sulfated, which decreases reabsorption and increases fecal excretion. Bile acids are absorbed passively in the epithelium of the small and large intestine and actively in the distal ileum.
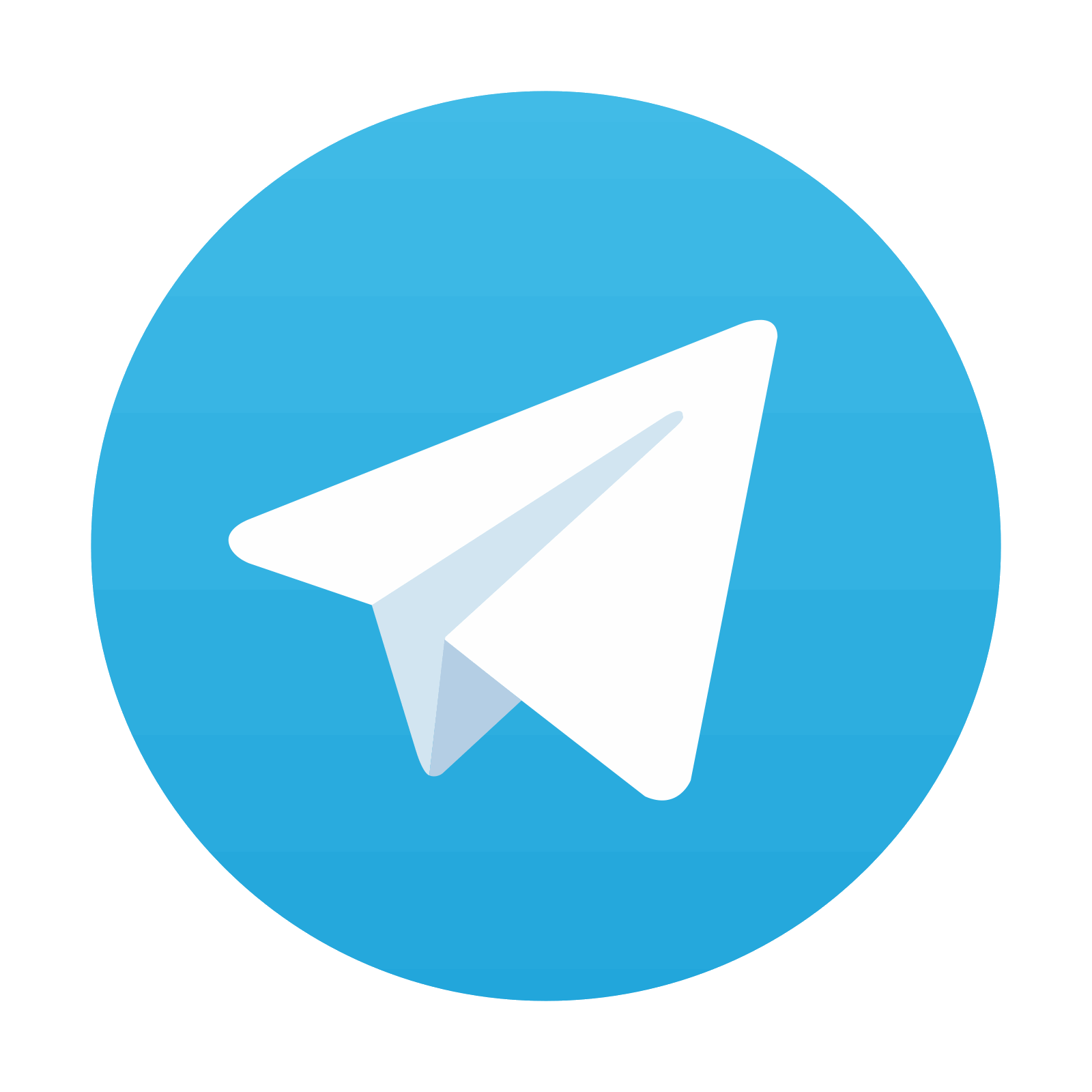
Stay updated, free articles. Join our Telegram channel
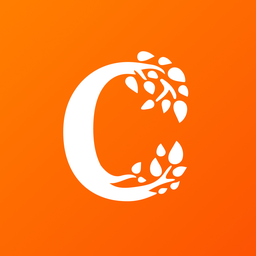
Full access? Get Clinical Tree
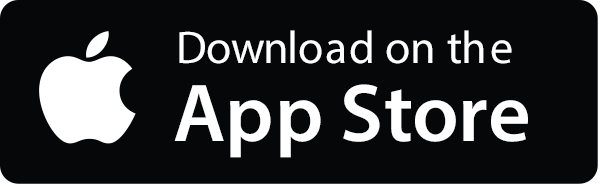
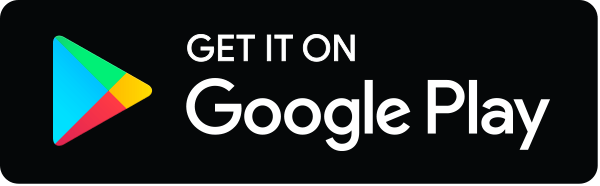