50
Plasma Proteins & Immunoglobulins
Robert K. Murray, MD, PhD, Molly Jacob, MB BS, MD, PhD, & Joe Varghese, MB BS, MD
OBJECTIVES
After studying this chapter, you should be able to:
List the major functions of blood.
Explain the functions of the major plasma proteins, including albumin, haptoglobin, transferrin, ceruloplasmin, α1-antitrypsin, and α2-macroglobulin.
Describe how iron homeostasis is maintained and how it is affected in certain disorders.
Describe the general structures and functions of the five classes of immunoglobulins and the uses of monoclonal antibodies.
Appreciate that the complement system is involved in a number of important biological processes.
Indicate the causes of Wilson disease, Menkes disease, the lung and liver diseases associated with α1-antitrypsin deficiency, amyloidosis, multiple myeloma, and agammaglobulinemia.
BIOMEDICAL IMPORTANCE
The fundamental role of blood in the maintenance of homeostasis (see Chapter 51) and the ease with which blood can be obtained have meant that the study of its constituents has been of central importance in the development of biochemistry and clinical biochemistry. The basic properties of a number of plasma proteins, including the immunoglobulins (antibodies), are described in this chapter. Changes in the amounts of various plasma proteins and immunoglobulins occur in many diseases and can be monitored by electrophoresis or other suitable procedures. As indicated in an earlier chapter, alterations of the activities of certain enzymes found in plasma are of diagnostic use in a number of pathologic conditions. Plasma proteins involved in blood coagulation are discussed in Chapter 51.
THE BLOOD HAS MANY FUNCTIONS
The functions of blood—except for specific cellular ones such as oxygen transport and cell-mediated immunologic defense—are carried out by plasma and its constituents (Table 50-1).
TABLE 50–1 Major Functions of Blood
Plasma consists of water, electrolytes, metabolites, nutrients, proteins, and hormones. The water and electrolyte composition of plasma is practically the same as that of all extracellular fluids. Laboratory determinations of levels of Na+, K+, Ca2+, Mg2+, Cl–, , PaCO2, and of blood pH are important in the management of many patients.
PLASMA CONTAINS A COMPLEX MIXTURE OF PROTEINS
The concentration of total protein in human plasma is approximately 7.0-7.5 g/dL and comprises the major part of the solids of the plasma. The proteins of the plasma are actually a complex mixture that includes not only simple proteins but also conjugated proteins such as glycoproteins and various types of lipoproteins. Use of proteomic techniques is allowing the isolation and characterization of previously unknown plasma proteins, some present in very small amounts (eg, detected in hemodialysis fluid and in the plasma of patients with cancer), thus expanding the plasma proteome. Thousands of antibodies are present in human plasma, although the amount of any one antibody is usually quite low under normal circumstances. The relative dimensions and molecular masses of some of the most important plasma proteins are shown in Figure 50–1.
FIGURE 50–1 Relative dimensions and approximate molecular masses of protein molecules in the blood (Oncley).
The separation of individual proteins from a complex mixture is frequently accomplished by the use of solvents or electrolytes (or both) to remove different protein fractions in accordance with their solubility characteristics. This is the basis of the so-called salting-out methods, which find some usage in the determination of protein fractions in the clinical laboratory. Thus, one can separate the proteins of the plasma into three major groups—fibrinogen, albumin, and globulins—by the use of varying concentrations of sodium or ammonium sulfate.
The most common method of analyzing plasma proteins is by electrophoresis. There are many types of electrophoresis, each using a different supporting medium. In clinical laboratories, cellulose acetate is widely used as a supporting medium. Its use permits resolution, after staining, of plasma proteins into five bands, designated albumin, α1, α2, β, and γ fractions, respectively (Figure 50–2). The stained strip of cellulose acetate (or other supporting medium) is called an electrophoretogram. The amounts of these five bands can be conveniently quantified by use of densitometric scanning machines. Characteristic changes in the amounts of one or more of these five bands are found in many diseases.
FIGURE 50–2 Technique of cellulose acetate zone electrophoresis. (A) A small amount of serum or other fluid is applied to a cellulose acetate strip. (B) Electrophoresis of sample in electrolyte buffer is performed. (C) Separated protein bands are visualized in characteristic positions after being stained. (D) Densitometer scanning from cellulose acetate strip converts bands to characteristic peaks of albumin, α1,-globulin, β2-globulin, β-globulin, and γ-globulin. (Reproduced, with permission, from Parslow TG et al (editors): Medical Immunology, 10th ed. McGraw-Hill, 2001.)
The Concentration of Protein in Plasma Is Important in Determining the Distribution of Fluid Between Blood & Tissues
In arterioles, the hydrostatic pressure is about 37 mmHg, with an interstitial (tissue) pressure of 1 mmHg opposing it. The osmotic pressure (oncotic pressure) exerted by the plasma proteins is approximately 25 mmHg. Thus, a net outward force of about 11 mmHg drives fluid out into the interstitial spaces. In venules, the hydrostatic pressure is about 17 mmHg, with the oncotic and interstitial pressures as described above; thus, a net force of about 9 mmHg attracts water back into the circulation. The above pressures are often referred to as the Starling forces. If the concentration of plasma proteins is markedly diminished (eg, due to severe protein malnutrition), fluid is not attracted back into the intravascular compartment and accumulates in the extravascular tissue spaces, a condition known as edema. Edema has many causes; protein deficiency is one of them.
Plasma Proteins Have Been Studied Extensively
Because of the relative ease with which they can be obtained, plasma proteins have been studied extensively in both humans and animals. Considerable information is available about the biosynthesis, turnover, structure, and functions of the major plasma proteins. Alterations of their amounts and of their metabolism in many disease states have also been investigated. In recent years, many of the genes for plasma proteins have been cloned and their structures determined.
The preparation of antibodies specific for the individual plasma proteins has greatly facilitated their study, allowing the precipitation and isolation of pure proteins from the complex mixture present in tissues or plasma. In addition, the use of isotopes has made possible the determination of their pathways of biosynthesis and of their turnover rates in plasma.
The following generalizations have emerged from studies of plasma proteins.
Most Plasma Proteins Are Synthesized in the Liver
This has been established by experiments at the whole animal level (eg, hepatectomy) and by use of the isolated perfused liver preparation, of liver slices, of liver homogenates, and of in vitro translation systems using preparations of mRNA extracted from liver. However, the γ-globulins are synthesized in plasma cells and certain plasma proteins are synthesized in other sites, such as endothelial cells.
Plasma Proteins Are Generally Synthesized on Membrane-Bound Polyribosomes
They then traverse the major secretory route in the cell (rough endoplasmic membrane → smooth endoplasmic membrane → Golgi apparatus → secretory vesicles) prior to entering the plasma. Thus, most plasma proteins are synthesized as preproteins and initially contain amino terminal signal peptides (Chapter 46). They are usually subjected to various posttranslational modifications (proteolysis, glycosylation, phosphorylation, etc) as they travel through the cell. Transit times through the hepatocyte from the site of synthesis to the plasma vary from 30 min to several hours or more for individual proteins.
Most Plasma Proteins Are Glycoproteins
Accordingly, they generally contain either N- or O-linked oligosaccharide chains, or both (Chapter 47). Albumin is the major exception; it does not contain sugar residues. The oligosaccharide chains have various functions (Table 47-2). Removal of terminal sialic acid residues from certain plasma proteins (eg, ceruloplasmin) by exposure to neuraminidase can markedly shorten their half-lives in plasma (Chapter 47).
Many Plasma Proteins Exhibit Polymorphism
A polymorphism is a Mendelian or monogenic trait that exists in the population in at least two phenotypes, neither of which is rare (ie, neither of which occurs with frequency of <1-2%). The ABO blood group substances (Chapter 52) are the best known examples of human polymorphisms. Human plasma proteins that exhibit polymorphism include α1– antitrypsin, haptoglobin, transferrin, ceruloplasmin, and immunoglobulins. The polymorphic forms of these proteins can be distinguished by different procedures (eg, various types of electrophoresis or isoelectric focusing), in which each form may show a characteristic migration. Analyses of these human polymorphisms have proved to be of genetic, anthropologic, and clinical interest.
Each Plasma Protein Has a Characteristic Half-Life in the Circulation
The half-life of a plasma protein can be determined by labeling the isolated pure protein with 131I or Cr51 under mild, nonde-naturing conditions. The labeled protein is freed of unbound free isotope and its specific activity (disintegrations per minute per milligram of protein) determined. A known amount of the radioactive protein is then injected into a normal adult subject, and samples of blood are taken at various time intervals for determinations of radioactivity. The values for radioactivity are plotted against time, and the half-life of the protein (the time for the radioactivity to decline from its peak value to one-half of its peak value) can be calculated from the resulting graph, discounting the times for the injected protein to equilibrate (mix) in the blood and in the extravascular spaces. The half-lives obtained for albumin and haptoglobin in normal healthy adults are approximately 20 and 5 days, respectively. In certain diseases, the half-life of a protein may be markedly altered. For instance, in some gastrointestinal diseases such as regional ileitis (Crohn’s disease), considerable amounts of plasma proteins, including albumin, may be lost into the bowel through the inflamed intestinal mucosa. Patients with this condition have a protein-losing gastroenteropathy, and the half-life of injected iodinated albumin in these subjects may be reduced to as little as 1 day.
The Levels of Certain Proteins in Plasma Increase During Acute Inflammatory States or Secondary to Certain Types of Tissue Damage
These proteins are called “acute-phase proteins” (or reactants) and include C-reactive protein (CRP, so named because it reacts with the C polysaccharide of pneumococci), α1-antitrypsin, haptoglobin, α1 acid glycoprotein, and fibrinogen. The elevations of the levels of these proteins vary from as little as 50% to as much as 1000-fold in the case of CRP. Their levels are also usually elevated during chronic inflammatory states and in patients with cancer. These proteins are believed to play a role in the body’s response to inflammation. For example, C-reactive protein can stimulate the classic complement pathway (see below), and α1-antitrypsin can neutralize certain proteases released during the acute inflammatory state. CRP is used as a marker of tissue injury, infection, and inflammation, and there is considerable interest in its use as a predictor of certain types of cardiovascular conditions secondary to atherosclerosis. The cytokine (= a protein made by cells that affects the behavior of other cells) interleukin-1 (IL-1), a poly-peptide released from mononuclear phagocytic cells, is the principal—but not the sole—stimulator of the synthesis of the majority of acute phase reactants by hepatocytes. Additional molecules such as IL-6 are involved, and they as well as IL-1 appear to work at the level of gene transcription.
Nuclear factor kappa-B (NFkB) is a transcription factor that has been involved in the stimulation of synthesis of certain of the acute phase proteins. This important factor is also involved in the expression of many cytokines, chemokines, growth factors, and cell adhesion molecules implicated in immunologic phenomena. Normally, it exists in an inactive form in the cytosol but is activated and translocated to the nucleus via the action of a number of molecules (eg, interleukin-1) produced in processes such as inflammation, infection, and radiation injury.
Table 50-2 summarizes the functions of many of the plasma proteins. The remainder of the material in this chapter presents basic information regarding selected plasma proteins: albumin, haptoglobin, transferrin, ceruloplasmin, α1-antitrypsin, α2-macroglobulin, the immunoglobulins, and the complement system. The lipoproteins are discussed in Chapter 25. New information is constantly forthcoming on plasma proteins and their variants (including those discussed here), as the techniques of proteomics, particularly sensitive new methods of determining proteins sequences by mass spectrometry (see Chapter 4), are applied to their study. A number of laboratories are participating in efforts to determine the complete human plasma protein proteome. It is believed that this will shed further light on genetic variations in humans and also provide many new biomarkers to aid in the diagnosis of many diseases. (A biomarker has been defined as a characteristic that is objectively measured and evaluated as an indicator of normal biologic processes, pathogenic processes, or pharmacologic responses to a therapeutic intervention.)
TABLE 50–2 Some Functions of Plasma Proteins
Albumin Is the Major Protein In Human Plasma
Albumin (69 kDa) is the major protein of human plasma (3.44.7 g/dL) and makes up approximately 60% of the total plasma protein. About 40% of albumin is present in the plasma, and the other 60% is present in the extracellular space. The liver produces about 12 g of albumin per day, representing about 25% of total hepatic protein synthesis and half its secreted protein. Albumin is initially synthesized as a preproprotein. Its signal peptide is removed as it passes into the cisternae of the rough endoplasmic reticulum, and a hexapeptide at the resulting amino terminal is subsequently cleaved off farther along the secretory pathway (see Figure 46–12). The synthesis of albumin is depressed in a variety of diseases, particularly those of the liver. The plasma of patients with liver disease often shows a decrease in the ratio of albumin to globulins (decreased albumin-globulin ratio). The synthesis of albumin decreases relatively early in conditions of protein malnutrition, such as kwashiorkor.
Mature human albumin consists of one polypeptide chain of 585 amino acids and contains 17 disulfide bonds. By the use of proteases, albumin can be subdivided into three domains, which have different functions. Albumin has an ellipsoidal shape, which means that it does not increase the viscosity of the plasma as much as an elongated molecule such as fibrinogen does. Because of its relatively low molecular mass (about 69 kDa) and high concentration, albumin is thought to be responsible for 75-80% of the osmotic pressure of human plasma. Electrophoretic studies have shown that the plasma of certain humans lacks albumin. These subjects are said to exhibit analbuminemia. One cause of this condition is a mutation that affects splicing. Subjects with analbuminemia show only moderate edema, despite the fact that albumin is the major determinant of plasma osmotic pressure. It is thought that the amounts of the other plasma proteins increase and compensate for the lack of albumin.
Another important function of albumin is its ability to bind various ligands. These include free fatty acids (FFA), calcium, certain steroid hormones, bilirubin, and some of the plasma tryptophan. In addition, albumin appears to play an important role in transport of copper in the human body (see below). A variety of drugs, including sulfonamides, penicillin G, dicumarol, and aspirin, are bound to albumin; this finding has important pharmacologic implications.
Preparations of human albumin have been widely used in the treatment of hemorrhagic shock and of burns. However, some recent studies question the value of this therapy.
Haptoglobin Binds Extracorpuscular Hemoglobin, Preventing Free Hemoglobin from Entering the Kidney
Haptoglobin (Hp) is a plasma glycoprotein that binds extracorpuscular hemoglobin (Hb) in a tight noncovalent complex (Hb-Hp). The amount of haptoglobin in human plasma ranges from 40 to 180 mg of hemoglobin-binding capacity per deciliter. Approximately 10% of the hemoglobin that is degraded each day is released into the circulation and is thus extracor-puscular. The other 90% is present in old, damaged red blood cells, which are degraded by cells of the histiocytic system. The molecular mass of hemoglobin is approximately 65 kDa, whereas the molecular mass of the simplest polymorphic form of haptoglobin (Hp 1-1) found in humans is approximately 90 kDa. Thus, the Hb-Hp complex has a molecular mass of approximately 155 kDa. Free hemoglobin passes through the glomerulus of the kidney, enters the tubules, and tends to precipitate therein (as can happen after a massive incompatible blood transfusion, when the capacity of haptoglobin to bind hemoglobin is grossly exceeded) (Figure 50–3). However, the Hb-Hp complex is too large to pass through the glomerulus. The function of Hp thus appears to prevent loss of free hemoglobin into the kidney. This conserves the valuable iron present in hemoglobin, which would otherwise be lost to the body.
FIGURE 50–3 Different fates of free hemoglobin and of the hemoglobin-haptoglobin complex.
Human haptoglobin exists in three polymorphic forms, known as Hp 1-1, Hp 2-1, and Hp 2-2. Hp 1-1 migrates in starch gel electrophoresis as a single band, whereas Hp 2-1 and Hp 2-2 exhibit much more complex band patterns. Two genes, designated Hp1 and Hp2, direct these three phenotypes, with Hp 2-1 being the heterozygous phenotype. It has been suggested that the haptoglobin polymorphism may be associated with the prevalence of many inflammatory diseases.
The levels of haptoglobin in human plasma vary and are of some diagnostic use. Low levels of haptoglobin are found in patients with hemolytic anemias. This is explained by the fact that whereas the half-life of haptoglobin is approximately 5 days, the half-life of the Hb-Hp complex is about 90 min, the complex being rapidly removed from plasma by hepatocytes. Thus, when haptoglobin is bound to hemoglobin, it is cleared from the plasma about 80 times faster than normally. Accordingly, the level of haptoglobin falls rapidly in situations where hemoglobin is constantly being released from red blood cells, such as occurs in hemolytic anemias. Haptoglobin is an acute phase protein, and its plasma level is elevated in a variety of inflammatory states.
Haptoglobin-related protein is another protein found in human plasma. It bears a high degree of homology to haptoglobin and it appears to bind hemoglobin. Its level is elevated in some patients with cancers, although the significance of this is not understood.
Certain other plasma proteins bind heme but not hemoglobin. Hemopexin is a β1-globulin that binds free heme. Albumin will bind some metheme (ferric heme) to form methemalbumin, which then transfers the metheme to hemopexin.
IRON IS A VERY IMPORTANT BODY CONSTITUENT AND IS ZEALOUSLY CONSERVED
Transferrin is an important plasma protein involved in iron transport. Before discussing it and a number of other proteins involved in iron homeostasis, we shall describe certain aspects of iron and its metabolism.
Iron is important in the human body because of its occurrence in many hemoproteins such as hemoglobin, myoglobin, and the cytochromes (including the cytochrome P450 group of enzymes). A normal 70 kg adult male has 3-4 g of iron in his body, the distribution of which is shown in Table 50-3. Under normal circumstances, the body guards its content of iron zealously. A healthy adult male loses only about 1 mg/day, which is replaced by absorption from the intestine. Adult females (premenopausal) require about 1.5 mg/day and are more prone to develop states of iron deficiency because of blood loss during menstruation.
TABLE 50–3 Distribution of Iron in a 70-kg Adult Male1
Absorption of Dietary Iron Occurs in the Duodenum
Iron is ingested in the diet either as non-heme or heme iron. Absorption of iron by enterocytes of the proximal duodenum is a highly regulated process (Figure 50–4). Inorganic dietary iron in the ferric state (Fe3+) is reduced to its ferrous form (Fe2+) by a brush border membrane-bound ferrireductase, duodenal cytochrome b (Dcytb). Vitamin C, gastric acid, and a number of other reducing agents present in food may also favor reduction of ferric to ferrous iron. The transfer of iron across the apical membrane of the enterocytes is accomplished via the divalent metal transporter 1 (DMT1 or SLC11A2). DMT1 is relatively non-specific and may also be involved in the transport of other divalent cations, such as Mn2+, Co2+, Zn2+, Cu2+, and Pb2+. Once inside the enterocytes, iron can either be stored as ferritin or transferred across the basolateral membrane into the circulation by the iron exporter protein, ferroportin or iron-regulated protein 1 (IREG1 or SLC40A1). This process occurs in conjunction with hephaestin, a copper-containing ferroxidase homologous to ceruloplasmin, which oxidizes Fe2+ to Fe3+. Iron is transported in plasma in the Fe3+ form by the transport protein, transferrin. Excess iron that is stored in the enterocytes as ferritin is lost when the enterocytes are sloughed off into the gut lumen.
FIGURE 50–4 Non-heme iron transport in enterocytes. Ferric iron is reduced to the ferrous form by a luminal ferrireductase, duodenal cytochrome b (Dcytb). Ferrous iron is transported into the enterocyte via divalent metal transporter-1 (DMT-1). Within the enterocyte, iron is either stored as ferritin, or transported out of the cell, across the baslolateral membrane, by ferroportin (Fp). Ferrous iron is oxidized to its ferric form by the ferroxidase, hephaestin. Ferric iron is then bound by transferrin in blood which transports it to various sites in the body. (Based on Andrews NC: Forging a field: the golden age of iron biology. Blood 2008;112(2);219.)
Heme iron in the diet is taken up by enterocytes by mechanisms independent of those involved in the uptake of dietary inorganic iron. Heme in enterocytes is broken down by heme oxygenase (HO, see Chapter 31) to release iron, which is either stored as ferritin or transported into circulation by ferroportin.
Transferrin Shuttles Iron to Sites Where It Is Needed
Free iron is extremely toxic because of its ability to catalyze the formation of harmful oxygen free radicals, via the Fenton reaction (Figure 50–5). In biological systems, iron is always bound to proteins in order to limit the generation of toxic radicals. In the plasma, iron is tightly bound to the plasma protein, transferrin (Tf), which plays a central role in transporting iron around the body to sites where it is needed. It is a β1 – globulin with a molecular mass of approximately 76 kDa. It is a glycoprotein and is synthesized in the liver. It transports iron in circulation to sites where iron is required (eg, the bone marrow). It has two high-affinity binding sites for Fe3+ iron. Transferrin, when bound to two atoms of iron, is called holotransferrin (Tf-Fe). The concentration of Tf in plasma is approximately 300 mg/dL. This amount of transferrin can bind a total of approximately 300 μg of iron (per deciliter of plasma). This represents the total iron-binding capacity (TIBC) of plasma. Normally, transferrin is about 30% saturated with iron. Transferrin saturation decreases to less than 16% during severe iron deficiency and may increase to more than 45% in iron overload conditions.
FIGURE 50–5 The Fenton reaction. Free iron is extremely toxic as it can catalyze the formation of hydroxyl radical (OH–) from hydrogen peroxide (see also Chapter 52). The hydroxyl radical is a transient but highly reactive species and can oxidize cellular macromolecules resulting in tissue damage.
Glycosylation of transferrin is impaired in congenital disorders of glycosylation (Chapter 47) and chronic alcoholism, resulting in increased circulating levels of carbohydrate-deficient transferrin (CDT). CDT can be measured by isoelectric focussing (IEF) and is used as a marker of chronic alcoholism.
The Transferrin Cycle Helps in Cellular Uptake of Iron
Transferrin receptor 1 (TfR1) is present on the surface of almost all cells, especially erythroid precursors in the bone marrow. Transferrin binds to these receptors and is internalized by receptor-mediated endocytosis (similar to LDL receptors described in Chapter 25). The acidic pH inside the late endosome causes iron to dissociate from transferrin (Tf). The dissociated iron leaves the endosome via DMT1 to enter the cytoplasm. Unlike the protein component of LDL, apoTf (Tf without iron bound to it) is not degraded within the endosome. Instead, it remains associated with its receptor and returns to the plasma membrane. It can then dissociate from its receptor, re-enter plasma, and pick up more iron to be delivered to cells. This is called the transferrin cycle (Figure 50–6).
FIGURE 50–6 The transferrin cycle. Holotransferrin (Tf-Fe) binds to transferrin receptor 1 (TfR1) present in clathrin-coated pits on the cell surface. The TfR1-Tf-Fe complex is endocytosed and endocytic vesicles fuse to form early endosomes. The early endosomes mature to late endosomes, which have an acidic pH inside. The low pH causes release of iron from its binding sites on transferrin. Apotransferrin (apo-Tf) remains bound to TfR1. Ferric iron is converted to its ferrous form by the ferrireductase, Steap 3. Ferrous iron is then transported into the cytosol via DMT1. The TfR1-apo-Tf complex is recycled back to the cell surface. At the cell surface, apo-Tf is released from TFR1. TfR1 then binds to new Tf-Fe. This completes the transferrin cycle. (Based on Figure 17–48 in Lodish H et al: Molecular Cell Biology, 4th ed. WH Freeman, 2000).
Transferrin receptor 2 (TfR2) is expressed primarily on the surface of hepatocytes and also in the crypt cells of the small intestine. It has a low affinity for Tf-Fe and does not appear to be involved in iron uptake by cells. It plays a role in sensing body iron stores in association with other proteins, as discussed later.
Iron in Senescent Erythrocytes Is Recycled by Macrophages
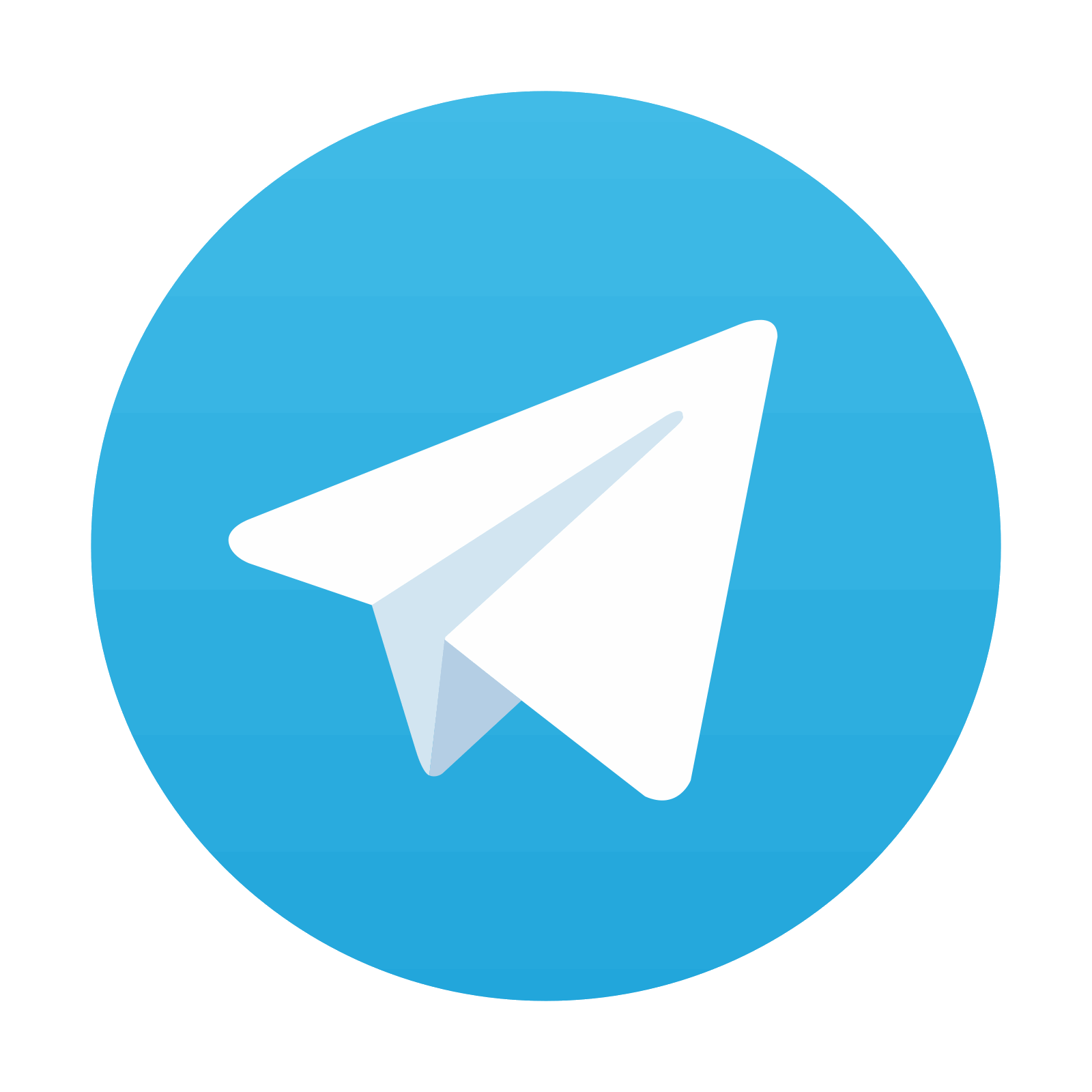
Stay updated, free articles. Join our Telegram channel
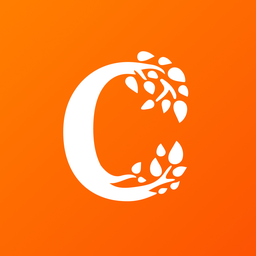
Full access? Get Clinical Tree
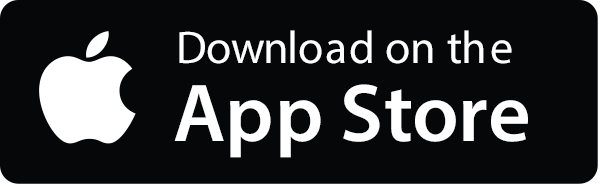
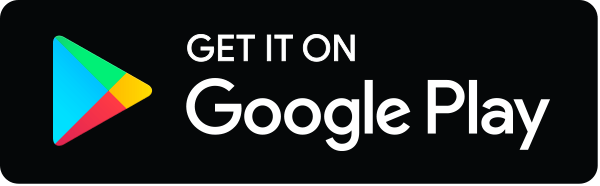