Fig. 16.1
Photomicrograph of a typical articular cartilage stained by hematoxylin and eosin staining. Articular cartilage can be subdivided into different zones from the cartilage surface to subchondral bone. In the superficial zone, collagen fibers are parallel arranged to cartilage surface and thus resistant to shear force. On the other hand, the radial zone can resist to compression, in which collagen fibers are vertical to the surface, and cells are arranged in columns. This zone contains the largest-diameter collagen fiber and the highest concentration of proteoglycans. Calcified cartilage acts as an anchor between articular cartilage and subchondral bone
Articular cartilage is referred to the hyaline cartilage on the articular surface of bones, which can bear a force up to six times the body weight and a stress approaching 10 MPa. Furthermore, the kinetic coefficient of friction for cartilage is less than 0.005 [2]. Even so, articular cartilage is frequently damaged by sport injury, trauma, or overuse. After damage, the cartilage has a limited ability for intrinsic repair because of its avascular and nearly acellular nature, which limits the ability of progenitor cells from the blood or bone marrow, and the resident articular chondrocytes that are entrapped within the surrounding matrix to migrate toward the injured tissue to secret the reparative matrix.
Traditional treatments for cartilage repair include allografts and autografts. There are many limitations for applications of cartilage grafts including donor’s availability, donor site injury, scarring, and pain. Allografts have a high risk of infection. In addition, the difficulty for implanted materials to integrate into the host tissue and the loss of cell viability due to graft storage often reduces the lifetime of the grafts. Autografts also suffer from the problem with integration and require additional defects to be created in the patients’ remaining cartilaginous tissue [1].
2 Tissue Engineering for Cartilage Repair Is an Open Challenge
Since cartilage has relatively poor self-healing capacity, preparation of functional biocartilage substitutes in vitro for cartilage repair is an attractive concept with the recent advances in tissue engineering. Tissue-engineering aims to combine three-dimensional (3D) scaffolds, cells, and bioactive factors to generate in vitro functional constructs for implantation. In the literature, regenerated cartilage can be derived from various cell types, including chondrocytes and mesenchymal stem cells (MSCs). Autologous chondrocyte implantation (ACI) is a cell-based therapy for repairing cartilage defects. Cartilage is taken from the lesser weight-bearing area of the bone interface. After removing the matrix, chondrocytes are isolated and cultured in vitro to obtain a large number of cells for transplantation. These fresh cells are harvested and injected on the damaged area in combination with a biomembrane to aid cell adhesion or preseeded in a scaffold matrix. Although autologous chondrocytes can reduce the risk of immune rejection, these are expanded cells and have a tendency to lose their phenotype in vitro [1, 3]. On the other hand, MSCs have the capacities for self-renewal, stemness maintenance, and the potential to differentiate into a variety of connective tissues including cartilage, bone, fat, tendon, and others. Therefore, MSCs have been extensively used as a cell source for cartilage regeneration [1, 4]. Recently, there are several kinds of strategies associated with the use of MSCs for cell-based therapies [5]. The first strategy is to inject the MSCs immediately into the damaged tissue where these donor cells are expected to participate in replacing the host cells. The second approach is to incorporate MSCs into 3D scaffolds to replace the damaged tissue. In the third concept, MSCs are considered as supporting cells that provide growth factors or cytokines to stimulate the reparative events in vivo [5].
A systematic review has examined the available clinical evidence to support the various MSC treatment strategies used in orthopedics for cartilage defect regeneration, based on the literature in PubMed database from 2002 to 2012 [6]. This systematic review showed an increasing number of published studies on this topic over time and identified 72 preclinical papers and 18 clinical trials. Among these, 50 preclinical studies and 11 clinical trials are associated with the use of cultured bone marrow-derived MSCs (BMSCs) [6]. BMSCs are the first identified and most studied adult MSCs and are commonly used as the cell source in many tissue-engineering fields. Owing to their well-characterized properties and differentiation potential, BMSCs are regarded as the “gold standard,” especially in cartilage and bone regeneration [7]. However, MSCs account for only 0.01–0.001 % of the nucleated cells in the adult human bone marrow [4]. Harvesting BMSCs is a painful and invasive procedure and may lead to complications. In addition, the cell number and differentiation capacities of human BMSCs are decreased with age [5, 8]. Hence, the use of autologous BMSCs for elderly patient in cartilage repair is significantly hindered.
3 Placental Stem Cells for Cartilage Tissue Engineering
More recently, MSCs have been isolated from extraembryonic tissues including amniotic fluid, umbilical cord, and placental. Because of the perinatal origin of these tissues, these MSCs are considered to be more primitive and could potentially be candidate cell sources for cartilage and bone regeneration [5]. The multilineage differentiation capacities of placenta-derived MSCs (PMSCs) have been reported, and lineages include osteogenesis, chondrogenesis, adipogenesis, neurogenesis, and endothelialization [9–13]. In addition, the immunosuppressive properties of PMSCs have been demonstrated to be significantly stronger than that of BMSCs, which further supports the consideration of PMSCs as a clinically viable source for cell therapy [14]. However, the number of studies associated with the application of human PMSCs (hPMSCs) in cartilage regeneration is rather limited when compared with those of BMSCs and umbilical cord-derived MSCs (UCMSCs).
The first study on evaluating the chondrogenic differentiation ability of hPMSCs was reported by Zhang et al. [13]. hPMSCs isolated from chorionic villi were pelleted into micromass and the chondrogenesis was induced by serum-free medium in the presence of BMP-2, TGF-β3, and dexamethasone. The pellets of cultured PMSC were similar in size and weight as compared with those of BMSC after 3 weeks of induction. The upregulation of cartilage-specific genes, including SOX9, COL2A1, aggrecan, COL10A1, BMP-2, and BMP-6, was detected in the pellets after induction, indicating that PMSCs had the potential for chondrogenic differentiation. To further examine the chondrogenic differentiation of PMSCs, cells were cultured in atelocollagen gel with the chondrogenic medium. The cell-atelocollagen gel became white, glistening, and harder than the original gel after 3 weeks of culture. Metachromatic territorial matrices were observed in the gel by the staining of toluidine blue, and cells with lacuna formation were also examined in the cartilage-like matrix. Moreover, PMSCs were seeded in the collagen sponges and implanted into the osteochondral defects of nude rats in vivo. Histological analyses showed that the reparative tissue filled the defect with cartilage-like surface and closely adhered to the residual part of the bone. Although the formation of hypertrophic repair cartilage was also observed at the top part of newly formed tissue, the bottom one had the strongest metachromasial staining and round cells, which indicated the formation of hyaline cartilage by PMSCs. The results of this study are encouraging and suggest that PMSCs can be one of the possible allogeneic cell sources for cartilage tissue engineering.
There are several studies that compared the chondrogenic potential of PMSCs with MSCs isolated from the other tissues [15–17]. In one study, MSCs from fetal and adult tissues were phenotypically characterized and examined for their differentiation capacities, based on morphological criteria and the expression of ECM components [15]. Fetal lung-derived MSCs (FLMSCs) and PMSCs showed a more elongated and thinner shape compared with the rounder and thicker shape of BMSCs as cultured on the tissue cultured polystyrene surface. The chondrogenic differentiation potential of MSCs was evaluated in pellet culture by induction with TGF-β3, BMP-6, and dexamethasone. Although MSCs from these four different sources could all form a pellet after centrifugation, the pellets of FLMSCs and PMSCs were frequently less stable and showed a more irregular shape compared with the pellets from fetal bone marrow (fBM) and adult bone marrow (aBM) MSCs that were firm and spherical. In addition, MSCs of bone marrow origin, either fetal or adult, exhibit better chondrogenesis than FLMSCs and PMSCs, judging by the appearance of typical morphological features of cartilage, the intensity of toluidine blue staining, and the expression of collagen type II, IX, and X after being cultured under chondrogenic conditions.
In another study, the morphology, immunophenotype, and differentiation patterns of MSCs isolated from the bone marrow, umbilical cord blood (UCB), and placenta were compared [16]. Three morphologically distinguishable cell types were indentified in cultures from BM (n = 9). The most common type observed was fibroblastoid cells, corresponding to 83 ± 7 % of all cells present in culture. In cultures of UCB-MSCs (n = 11) and PMSCs (n = 5), the same three morphologically distinguishable cell types were observed, and fibroblastoid cells remained the most frequent (85 ± 6 % and 86 ± 5 %, respectively). Although the morphology was similar, a distinct type of cartilage formation was observed in pelleted micromasses from BMSCs and PMSCs compared with UCB-MSCs as evidenced by alcian blue staining after chondrogenic induction for 4 weeks. It was observed that in pelleted micromasses from BMSC and PMSC samples, cells grouped into small clusters located in the same area, a feature typical of hyaline cartilage. In contrast, pelleted micromasses from UCB-MSC showed minimal matrix formation and a predominance of fibrous collagen deposition, which indicates the presence of fibrocartilage formation.
In an attempt to establish the relative potential of alternative MSCs sources, Cavallo et al. analyzed and compared the yield and differentiation potential of human MSCs from adipose tissue (AT) and BM tissues of cadaveric origins, and from fetal annexes (placenta and umbilical cord) after delivery using standardized isolation and culture protocols [17]. After MSC selection through tissue culture plastic adhesion, cells displayed a comparable proliferation capacity with distinct colony morphologies and were positive for a pool of typical MSCs markers. To successfully isolated MSCs through adherence selection, a minimum cell density of 1 × 105 cells/cm2 was necessary for mononuclear cells (MNCs) derived from AT, amniotic, and chorionic membranes of placenta. As for the MNCs from the mucous connective tissue of UC called Wharton’s Jelly, the cell density had to be doubled to achieve MSC colony formation. On the other hand, the minimum cell density required to isolate MSCs from the BM was 5 × 105 cells/cm2, thus implying a relative lower amount of MSCs present in BM and UC compared to AT, amniotic and chorionic membranes. Moreover, MSCs from fetal annexes had the tendency to form better-defined colonies than MSCs from BM or AT. In particular, MSCs from the chorionic membrane and in part MSCs derived from the Wharton’s Jelly seemed to continuously grow in confined colonies, suggesting that these cells may have a more primitive phenotype and a higher potency likely due to developmental-age differences. In the in vitro differentiation assay, cells were plated in six well plates at a density of 1 × 105 cells/cm2 and induced by osteogenic or chondrogenic medium. Results showed a higher osteogenic differentiation capacity of AT- and BM-derived MSCs, and a higher chondrogenic differentiation capacity of BM-derived MSCs. The expression of chondrogenic marker genes (e.g., SOX9, aggrecan, and type II collagen) for MSCs isolated from amniotic or chorionic membranes of placenta was significantly lower than those of BM-derived MSCs.
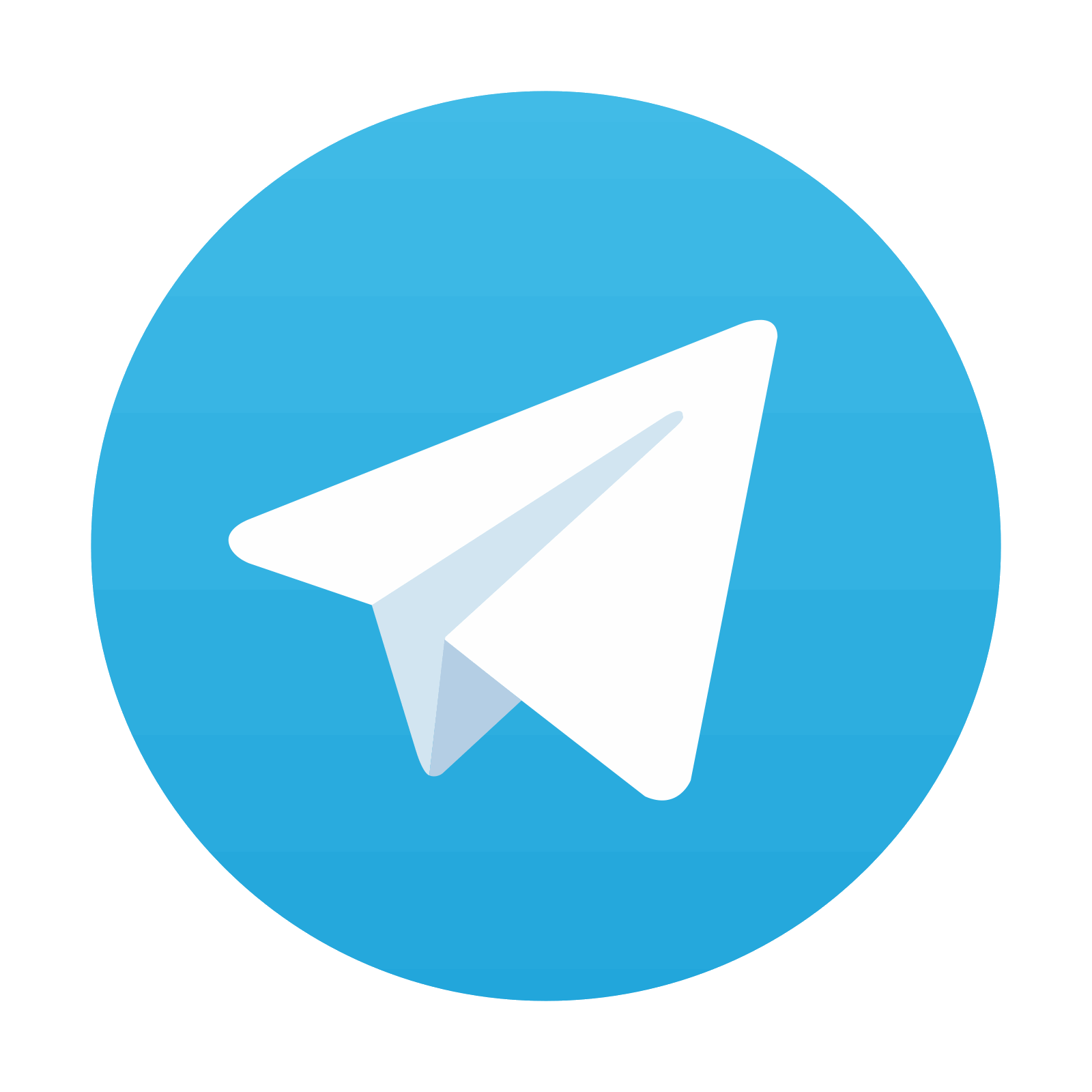
Stay updated, free articles. Join our Telegram channel
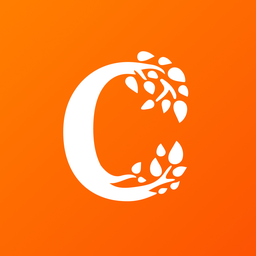
Full access? Get Clinical Tree
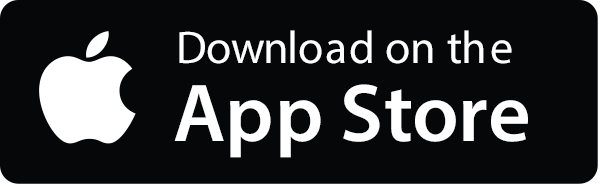
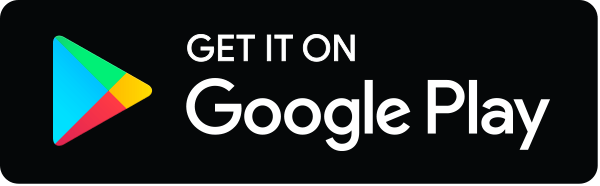