Energy from fuel oxidation is converted to the high-energy phosphate bonds of adenosine triphosphate (ATP) by the process of oxidative phosphorylation. Most of the energy from oxidation of fuels in the tricarboxylic acid (TCA) cycle and other pathways is conserved in the form of the reduced electron-accepting coenzymes, NADH and FAD(2H). The electron transport chain oxidizes NADH and FAD(2H) and donates the electrons to O2, which is reduced to H2O (Fig. 24.1). Energy from reduction of O2 is used for phosphorylation of adenosine diphosphate (ADP) to ATP by ATP synthase (F0F1ATPase). The net yield of oxidative phosphorylation is ~2.5 mol of ATP per mole of NADH oxidized or 1.5 mol of ATP per mole of FAD(2H) oxidized.
FIGURE 24.1 Oxidative phosphorylation. Red arrows show the path of electron transport from NADH to O2. As electrons pass through the chain, protons are pumped from the mitochondrial matrix to the intermembrane space, thereby establishing an electrochemical potential gradient, Δp, across the inner mitochondrial membrane. The positive and negative charges on the membrane denote the membrane potential (Δψ). Δp drives protons into the matrix through a pore in ATP synthase, which uses the energy to form ATP from ADP and Pi. ADP, adenosine diphosphate; ATP, adenosine triphosphate; NAD, nicotinamide adenine dinucleotide; Pi, inorganic phosphate.
Chemiosmotic Model of ATP Synthesis. The chemiosmotic model explains how energy from transport of electrons to O2 is transformed into the high-energy phosphate bonds of ATP (see Fig. 24.1). Basically, the electron transport chain contains three large protein complexes (I, III, and IV) that span the inner mitochondrial membrane. As electrons pass through these complexes in a series of oxidation–reduction reactions, protons are transferred from the mitochondrial matrix to the cytosolic side of the inner mitochondrial membrane. The pumping of protons generates an electrochemical gradient (Δp) across the membrane composed of the membrane potential and the proton gradient. ATP synthase contains a proton pore that spans the inner mitochondrial membrane and a catalytic headpiece that protrudes into the matrix. As protons are driven into the matrix through the pore, they change the conformation of the headpiece, which releases ATP from one site and catalyzes formation of ATP from ADP and inorganic phosphate (Pi) at another site.
Deficiencies of Electron Transport. In cells, complete transfer of electrons from NADH and FAD(2H) through the chain to O2 is necessary for ATP generation. Impaired transfer through any complex can have pathological consequences. Fatigue can result from iron-deficiency anemia, which decreases Fe for Fe–S centers and cytochromes. Cytochrome c1 oxidase, which contains the O2-binding site, is inhibited by cyanide. Mitochondrial DNA (mtDNA), which is maternally inherited, encodes some of the subunits of the electron transport chain complexes and ATP synthase. OXPHOS diseases are caused by mutations in nuclear DNA or mtDNA that decrease mitochondrial capacity for oxidative phosphorylation.
Regulation of Oxidative Phosphorylation. The rate of the electron transport chain is coupled to the rate of ATP synthesis by the transmembrane electrochemical gradient. As ATP is used for energy-requiring processes and ADP levels increase, proton influx through the ATP synthase pore generates more ATP, and the electron transport chain responds to restore Δp. In uncoupling, protons return to the matrix by a mechanism that bypasses the ATP synthase pore, and the energy is released as heat. Proton leakage, chemical uncouplers, and regulated uncoupling proteins increase our metabolic rate and heat generation.
Mitochondria and Cell Death. Although oxidative phosphorylation is a mitochondrial process, most ATP utilization occurs outside of the mitochondrion. ATP synthesized from oxidative phosphorylation is actively transported from the matrix to the intermembrane space by adenine nucleotide translocase (ANT). Porins form voltage-dependent anion channels (VDACs) through the outer mitochondrial membrane for the diffusion of H2O, ATP metabolites, and other ions. Under certain types of stress, ANT, VDACs, and other proteins form a nonspecific open channel known as the mitochondrial permeability transition pore. This pore is associated with events that lead rapidly to necrotic cell death.
THE WAITING ROOM 
Cora N. was recovering uneventfully from her heart attack 1 month earlier (see Chapter 20) when she won the Georgia state lottery. When she heard her number announced over television, she experienced crushing chest pain again and grew short of breath. Her family called 911 and she was rushed to the hospital emergency department.
On initial examination, her blood pressure was extremely high and her heart rhythm irregular. Cora N. was experiencing yet another myocardial infarction. An electrocardiogram showed unequivocal evidence of severe lack of oxygen (ischemia) in the muscles of the anterior and lateral walls of her heart. She was given nasal oxygen, and an intravenous drip of nitroglycerin, a vasodilating agent, was started in an effort to reduce her hypertension (it will also help to decrease her “preload” by vasodilating the vessels going to the heart). At this time, she did not show signs of systolic heart failure, so she was also given a β-blocker, which will help decrease her blood pressure as well as decrease the work of her heart by slowing her heart rate. After her blood pressure was well controlled, and because the hospital did not have a cardiac catheterization laboratory and a transfer to a hospital with a cath lab was not possible, a decision was made to administer intravenous tissue plasminogen activator (TPA) in an attempt to break up any intracoronary artery blood clots in vessels supplying the ischemic myocardium (thrombolytic therapy).
Stanley T. A 123I thyroid uptake and scan performed on Stanley T. confirmed that his hyperthyroidism was the result of Graves disease (see Chapter 20). Graves disease is an autoimmune genetic disorder caused by the generation of human thyroid-stimulating immunoglobulins. These immunoglobulins stimulate the growth of the thyroid gland (goiter) and excess secretion of the thyroid hormones, T3 and T4. Because heat production is increased under these circumstances, his heat intolerance and sweating were growing worse with time.
Isabel S., who has opioid use disorder and injects opioids intravenously, appeared to be responding well to her multidrug regimens to treat pulmonary tuberculosis and human immunodeficiency virus (HIV) (see Chapters 11, 12, 13, and 16). In the past 6 weeks, however, she has developed increasing weakness in her extremities, to the point that she has difficulty carrying light objects or walking. Physical examination indicates a diffuse proximal and distal muscle weakness associated with muscle atrophy. The muscles are not painful on motion but mildly tender to palpation. The blood level of the muscle enzymes, creatine phosphokinase (CK), and aldolase are elevated. An electromyogram (EMG) revealed a generalized reduction in the muscle action potentials, suggestive of a primary myopathic process. Proton spectroscopy of her brain and upper spinal cord showed no anatomic or biochemical abnormalities. The diffuse and progressive skeletal muscle weakness was out of proportion to that expected from her HIV or tuberculosis. This information led her physicians to consider other etiologies.
I. Oxidative Phosphorylation
Generation of ATP from oxidative phosphorylation requires an electron donor (NADH or FAD[2H]), an electron acceptor (O2), an intact inner mitochondrial membrane that is impermeable to protons, all the components of the electron transport chain, and ATP synthase. It is regulated by the rate of ATP utilization.
Most cells are dependent on oxidative phosphorylation for ATP homeostasis. During oxygen deprivation from ischemia (low blood flow), an inability to generate energy from the electron transport chain results in increased permeability of the inner mitochondrial membrane and mitochondrial swelling. Mitochondrial swelling is a key element in the pathogenesis of irreversible cell injury leading to cell lysis and death (necrosis).
A. Overview of Oxidative Phosphorylation
Our understanding of oxidative phosphorylation is based on the chemiosmotic hypothesis, which proposes that the energy for ATP synthesis is provided by an electrochemical gradient across the inner mitochondrial membrane. This electrochemical gradient is generated by the components of the electron transport chain, which pump protons across the inner mitochondrial membrane as they sequentially accept and donate electrons (see Fig. 24.1). The final acceptor is O2, which is reduced to H2O.
1. Electron Transfer from NADH to O2
In the electron transport chain, electrons donated by NADH or FAD(2H) are passed sequentially through a series of electron carriers embedded in the inner mitochondrial membrane. Each of the components of the electron transfer chain is reduced as it accepts an electron and then oxidized as it passes the electrons to the next member of the chain. From NADH, electrons are transferred sequentially through NADH:CoQ oxidoreductase (complex I, also known as NADH dehydrogenase), coenzyme Q (CoQ), the cytochrome b–c1 complex (complex III), cytochrome c, and finally cytochrome c oxidase (complex IV). NADH:CoQ oxidoreductase, the cytochrome b–c1 complex, and cytochrome c oxidase are multisubunit protein complexes that span the inner mitochondrial membrane. CoQ is a lipid-soluble quinone that is not protein bound and is free to diffuse in the lipid membrane. It transports electrons from complex I to complex III and is an intrinsic part of the proton pump for each of these complexes. Cytochrome c is a small protein in the intermembrane space that transfers electrons from the b–c1 complex to cytochrome oxidase. The terminal complex, cytochrome c oxidase, contains the binding site for O2. As O2 accepts electrons from the chain, it is reduced to H2O.
2. The Electrochemical Potential Gradient
At each of the three large membrane-spanning complexes in the chain, electron transfer is accompanied by proton pumping across the membrane. There is an energy drop of ~16 kcal in reduction potential as electrons pass through each of these complexes, which provides the energy required to move protons against a concentration gradient. The membrane is impermeable to protons, so they cannot diffuse through the lipid bilayer back into the matrix. Thus, in actively respiring mitochondria, the intermembrane space and cytosol may be ~0.75 pH unit lower than the matrix.
The transmembrane movement of protons generates an electrochemical gradient with two components: the membrane potential (the external face of the membrane is charged positive relative to the matrix side) and the proton gradient (the intermembrane space has a higher proton concentration and is, therefore, more acidic than the matrix) (Fig. 24.2). The electrochemical gradient is sometimes called the proton motive force because it is the energy that pushes the protons to reenter the matrix to equilibrate on both sides of the membrane. The protons are attracted to the more negatively charged matrix side of the membrane, where the pH is more alkaline.
FIGURE 24.2 Proton motive force (electrochemical gradient) across the inner mitochondrial membrane. The proton motive force consists of a membrane potential, Δψ, and a proton gradient, denoted by ΔpH for the difference in pH across the membrane. The electrochemical potential is called the proton motive force because it represents the potential energy of driving protons to return to the more negatively charged alkaline matrix.
3. ATP synthase
ATP synthase (F0F1ATPase), the enzyme that generates ATP, is a multisubunit enzyme that contains an inner membrane portion (F0) and a stalk and headpiece (F1) that project into the matrix (Fig. 24.3). The 12 c subunits in the membrane form a rotor that is attached to a central asymmetric shaft composed of the ε and γ subunits. The headpiece is composed of three αβ subunit pairs. Each β subunit contains a catalytic site for ATP synthesis. The headpiece is held stationary by a δ subunit attached to a long b subunit connected to subunit a in the membrane.
FIGURE 24.3 ATP synthase (F0F1ATPase). Note that the matrix side of the mitochondrial inner membrane is at the top of the figure. ADP, adenosine diphosphate; ATP, adenosine triphosphate; Pi, inorganic phosphate.
The influx of protons through the proton channel turns the rotor. The proton channel is formed by the c subunits on one side and the a subunits on the other side. Although the channel is continuous, it has two offset portions: one portion opens directly to the intermembrane space and one portion opens directly to the matrix. In the current model, each c subunit contains a glutamyl carboxyl group that extends into the proton channel. Because this carboxyl group accepts a proton from the intermembrane space, the c subunit rotates into the hydrophobic lipid membrane. The rotation exposes a different proton-containing c subunit to the portion of the channel that is open directly to the matrix side. Because the matrix has a lower proton concentration, the glutamyl carboxylic acid group releases a proton into the matrix portion of the channel. Rotation is completed by an attraction between the negatively charged glutamyl residue and a positively charged arginyl group on the a subunit.
According to the binding change mechanism, as the asymmetric shaft rotates to a new position, it forms different binding associations with the αβ subunits (Fig. 24.4). The new position of the shaft alters the conformation of one β subunit so that it releases a molecule of ATP and another subunit spontaneously catalyzes synthesis of ATP from inorganic phosphate, one proton, and ADP. Thus, energy from the electrochemical gradient is used to change the conformation of the ATP synthase subunits so that the newly synthesized ATP is released.
FIGURE 24.4 Binding change mechanism for ATP synthesis. The three αβ-subunit pairs of the ATP synthase headpiece have binding sites that can exist in three different conformations, depending on the position of the γ-stalk subunit. (1) When ADP + Pi bind to an open site and the proton influx rotates the γ-spindle (white arrow), the conformation of the subunits change and ATP is released from one site. (ATP dissociation is thus the energy-requiring step). Bound ADP and Pi combine to form ATP at another site. (2) As the ADP + Pi bind to the new open site, and the γ-shaft rotates, the conformations of the sites change again, and ATP is released. ADP and Pi combine to form another ATP. ADP, adenosine diphosphate; ATP, adenosine triphosphate; Pi, inorganic phosphate.
B. Oxidation–Reduction Components of the Electron Transport Chain
Electron transport to O2 occurs via a series of oxidation–reduction steps in which each successive component of the chain is reduced as it accepts electrons and oxidized as it passes electrons to the next component of the chain. The oxidation–reduction components of the chain include flavin mononucleotide (FMN), Fe–S centers, CoQ, and Fe in cytochromes b, c1, c, a, and a3. Copper (Cu) is also a component of cytochrome c oxidase (Fig. 24.5). With the exception of CoQ, all of these electron acceptors are tightly bound to the protein subunits of the carriers. FMN, like FAD, is synthesized from the vitamin riboflavin (see Fig. 23.4).
FIGURE 24.5 Components of the electron transport chain. NADH:CoQ oxidoreductase (complex I) spans the membrane and has a proton pumping mechanism involving CoQ. The electrons go from CoQ to the cytochrome b–c1 complex (complex III); electron transfer does not involve complex II. Succinate dehydrogenase (complex II), glycerol 3-phosphate dehydrogenase, and ETF:CoQ oxidoreductase all transfer electrons to CoQ, but they do not span the membrane and do not have proton pumping mechanisms. As CoQ accepts electrons and protons from the matrix side, it is converted to CoQH2. Electrons are transferred from complex III to complex IV (cytochrome c oxidase) by cytochrome c, a small cytochrome in the intermembrane space that has reversible binding sites on the b–c1 complex and cytochrome c oxidase. CoQ, coenzyme Q; ETF, electron-transferring flavoprotein; FMN, flavin mononucleotide; NAD, nicotinamide adenine dinucleotide.
The reduction potential of each complex of the chain is at a lower energy level than the previous complex, so energy is released as electrons pass through each complex. This energy is used to move protons against their concentration gradient, so they become concentrated on the cytosolic side of the inner membrane.
1. NADH:CoQ Oxidoreductase
NADH:CoQ oxidoreductase (also named NADH dehydrogenase) is an enormous 45-subunit complex that contains a binding site for NADH, several FMN, and iron–sulfur (Fe–S) center binding proteins, and binding sites for CoQ (see Fig. 24.5). An FMN accepts two electrons from NADH and is able to pass single electrons to the Fe–S centers. Fe–S centers, which are able to delocalize single electrons into large orbitals, transfer electrons to and from CoQ. Fe–S centers are also present in other enzyme systems, such as proteins within the cytochrome b–c1 complex, which transfer electrons to CoQ, and in aconitase in the TCA cycle.
2. Succinate Dehydrogenase and Other Flavoproteins
In addition to NADH:CoQ oxidoreductase, succinate dehydrogenase and other flavoproteins in the inner mitochondrial membrane also pass electrons to CoQ (see Fig. 24.5). Succinate dehydrogenase is part of the TCA cycle and also a component of complex II of the electron transport chain. ETF-CoQ oxidoreductase accepts electrons from ETF (electron-transferring flavoprotein), which acquires them from fatty acid oxidation and other pathways. Both of these flavoproteins have Fe–S centers. Glycerol 3-phosphate dehydrogenase is a flavoprotein that is part of a shuttle for reoxidizing cytoplasmic NADH (see Section I.E).
The free energy drop in electron transfer between NADH and CoQ of ~−13 to −14 kcal is able to support movement of four protons. However, the FAD in succinate dehydrogenase (as well as ETF-CoQ oxidoreductase and glycerol 3-phosphate dehydrogenase) is at roughly the same redox potential as CoQ, and no energy is released as they transfer electrons to CoQ. These proteins do not span the membrane and consequently do not have a proton pumping mechanism.
3. Coenzyme Q
CoQ is the only component of the electron transport chain that is not protein bound. The large hydrophobic side chain of 10 isoprenoid units (50 carbons) confers lipid solubility, and CoQ is able to diffuse freely through the lipids of the inner mitochondrial membrane (Fig. 24.6). When the oxidized quinone form accepts a single electron (to form the semiquinone), it forms a free radical (a compound with a single electron in an orbital). The transfer of single electrons makes it the major site for the generation of toxic oxygen free radicals in the body (see Chapter 25).
FIGURE 24.6 Structure of coenzyme Q. Coenzyme Q contains a quinone with a long lipophilic side chain comprising 10 isoprenoid units (thus, it is sometimes called CoQ10). CoQ can accept one electron (e−) to become the half-reduced form or two e−s to become fully reduced.
The semiquinone can accept a second electron and two protons from the matrix side of the membrane to form the fully reduced quinone. The mobility of CoQ in the membrane, its ability to accept one or two electrons, and its ability to accept and donate protons enable it to participate in the proton pumps for both complexes I and III as it shuttles electrons between them (see Section I.C). CoQ is also called ubiquinone (the ubiquitous quinone), because quinones with similar structures are found in all plants and animals.
4. Cytochromes
The remaining components in the electron transport chain are cytochromes (see Fig. 24.5). Each cytochrome is a protein that contains a bound heme (i.e., an Fe atom bound to a porphyrin nucleus similar in structure to the heme in hemoglobin) (Fig. 24.7).
FIGURE 24.7 Heme A. Heme A is found in cytochromes a and a3. Cytochromes are proteins that contain a heme chelated with an iron atom. Hemes are derivatives of protoporphyrin IX. Each cytochrome has a heme with different modifications of the side chains (indicated with dashed lines), resulting in a slightly different reduction potential and, consequently, a different position in the sequence of electron transfer.
Because of differences in the protein component of the cytochromes and small differences in the heme structure, each heme has a different reduction potential. The cytochromes of the b–c1 complex have a higher energy level than those of cytochrome oxidase (a and a3). Thus, energy is released by electron transfer between complexes III and IV. The iron atoms in the cytochromes are in the Fe3+ state. As they accept an electron, they are reduced to Fe2+. As they are reoxidized to Fe3+, the electrons pass to the next component of the electron transport chain.
5. Copper (Cu1) and the Reduction of Oxygen
The last cytochrome complex is cytochrome oxidase, which passes electrons from cytochrome c to O2 (see Fig. 24.5). It contains cytochromes a and a3 and the oxygen-binding site. A whole oxygen molecule, O2, must accept four electrons to be reduced to two H2O molecules. Bound copper (Cu+) ions in the cytochrome oxidase complex facilitate the collection of the four electrons and the reduction of O2.
Cytochrome oxidase has a much lower Km for O2 than myoglobin (the heme-containing intracellular oxygen carrier) or hemoglobin (the heme-containing oxygen transporter in the blood). Thus, O2 is “pulled” from the erythrocyte to myoglobin and from myoglobin to cytochrome oxidase, where it is reduced to H2O.
C. Pumping of Protons
One of the tenets of the chemiosmotic theory is that energy from the oxidation–reduction reactions of the electron transport chain is used to transport protons from the matrix to the intermembrane space. This proton pumping is generally facilitated by the vectorial arrangement of the membrane-spanning complexes. Their structure allows them to pick up electrons and protons on one side of the membrane and release protons on the other side of the membrane as they transfer an electron to the next component of the chain. The direct physical link between proton movement and electron transfer can be illustrated by an examination of the Q cycle for the b–c1 complex (Fig. 24.8). The Q cycle involves a double cycle of CoQ reduction and oxidation. CoQ accepts two protons at the matrix side together with two electrons; it then releases protons into the intermembrane space while donating one electron back to another component of the cytochrome b–c1 complex and one to cytochrome c.
FIGURE 24.8 The proton motive Q cycle for the b–c1 complex. (1) From 2QH2, electrons go down two different paths: One path is through an Fe–S center protein (ISP) toward cytochrome c (red arrows). Another path is “backward” to one of the b cytochromes (dashed arrows). (2) Electrons are transferred from ISP through cytochrome c1. Cytochrome c, which is in the intermembrane space, binds to the b–c1 complex to accept an electron. (3) Returning electrons go through another b cytochrome and are directed toward the matrix. (4) At the matrix side, electrons and 2H+ are accepted by Q. Q, coenzyme Q; Q•–, CoQ semiquinone; QH2, CoQ hydroquinone.
The mechanism for pumping protons at the NADH:CoQ oxidoreductase complex is not well understood, but it involves a Q cycle in which the Fe–S centers and FMN might participate. However, transmembrane proton movement at cytochrome c oxidase probably involves direct transport of the proton through a series of bound water molecules or amino acid side chains in the protein complex, a mechanism that has been described as a “proton wire.”
The significance of the direct link between the electron transfer and proton movement is that one cannot occur without the other (the processes are said to be “coupled”). Thus, when protons are not being used for ATP synthesis, the proton gradient and the membrane potential build up. This “proton back pressure” controls the rate of proton pumping, which controls electron transport and O2 consumption.
D. Energy Yield from the Electron Transport Chain
The overall free energy release from oxidation of NADH by O2 is ~−53 kcal, and from FAD(2H), it is ~−41 kcal. This ΔG0 is so negative that the chain is never reversible; we never synthesize oxygen from H2O. The negative ΔG0 also drives NADH and FAD(2H) formation from the pathways of fuel oxidation, such as the TCA cycle and glycolysis, to completion.
Overall, each NADH donates two electrons, equivalent to the reduction of one half of an O2 molecule. A generally (but not universally) accepted estimate of the stoichiometry of ATP synthesis is that four protons are pumped at complex I, four protons at complex III, and two at complex IV. With three protons translocated for each ATP synthesized and one proton for each phosphate transported into the matrix (see Section IV.A), an estimated 2.5 ATPs are formed for each NADH oxidized, and 1.5 ATPs are formed for each of the other FAD(2H)-containing flavoproteins that donate electrons to CoQ. (This calculation neglects the basal proton leak.) Thus, only ~30% of the energy available from NADH and FAD(2H) oxidation by O2 is used for ATP synthesis. Some of the remaining energy in the electrochemical potential is used for the transport of anions and Ca2+ into the mitochondrion. The remainder of the energy is released as heat. Consequently, the electron transport chain is also our major source of heat.
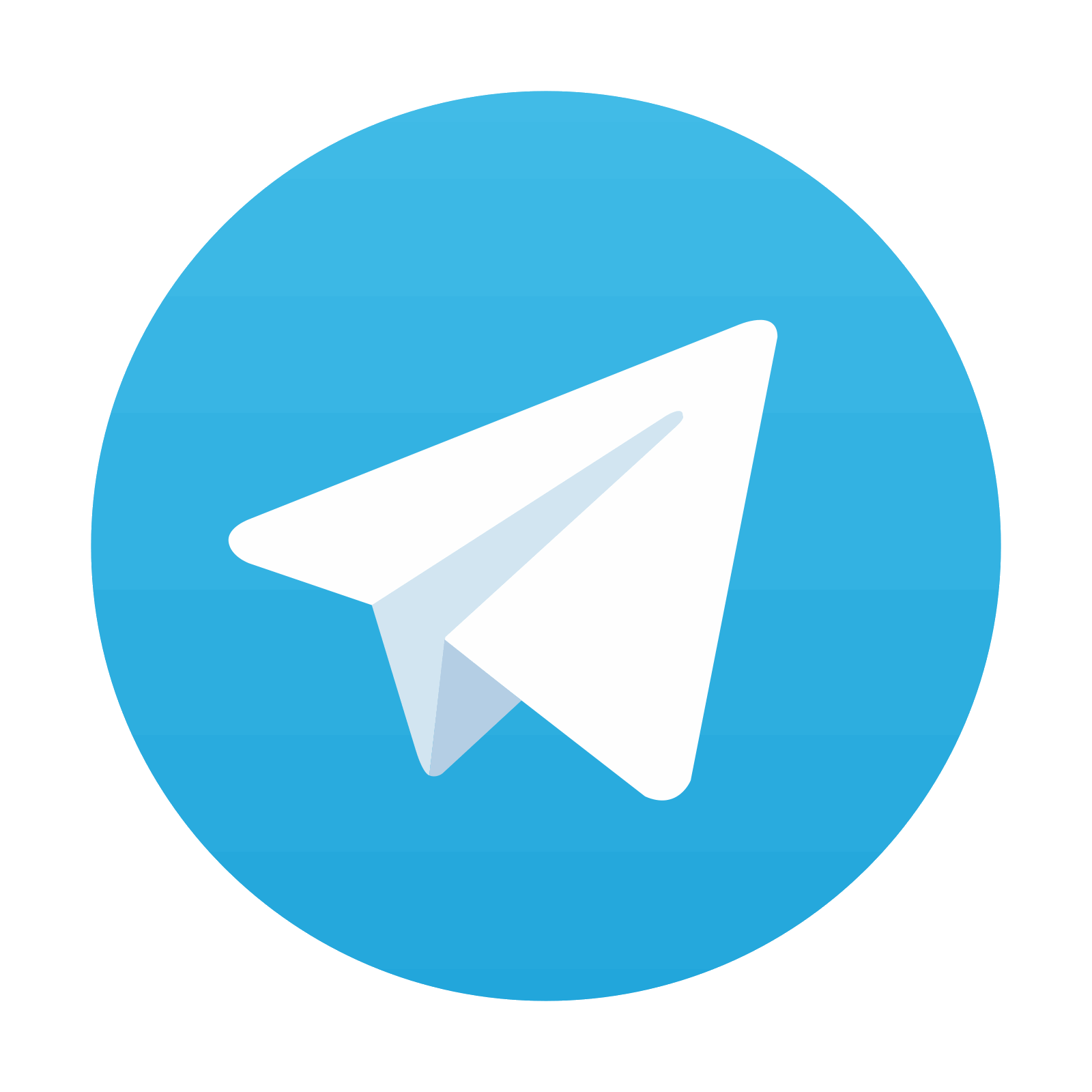
Stay updated, free articles. Join our Telegram channel
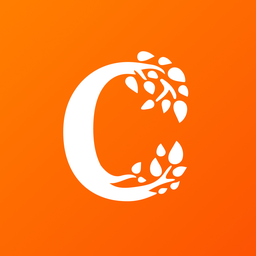
Full access? Get Clinical Tree
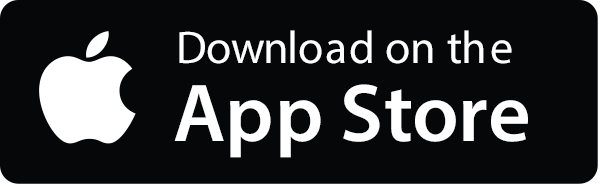
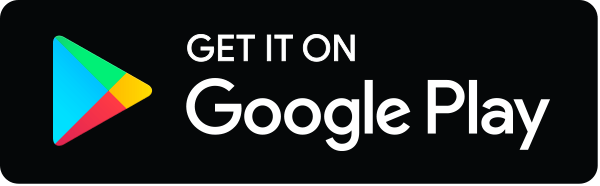