Fatty acids are a major fuel for humans and supply our energy needs between meals and during periods of increased demand, such as exercise. During overnight fasting, fatty acids become the major fuel for cardiac muscle, skeletal muscle, and liver. The liver converts fatty acids to ketone bodies (acetoacetate and β-hydroxybutyrate), which also serve as major fuels for tissues (e.g., the gut). The brain, which does not have a significant capacity for fatty acid oxidation, can use ketone bodies as a fuel during prolonged fasting.
The route of metabolism for a fatty acid depends somewhat on its chain length. Fatty acids are generally classified as very-long-chain length fatty acids (>C20), long-chain fatty acids (C12 to C20), medium-chain fatty acids (C6 to C12), and short-chain fatty acids (C4).
ATP is generated from oxidation of fatty acids in the pathway of β-oxidation. Between meals and during overnight fasting, long-chain fatty acids are released from adipose tissue triacylglycerols. They circulate through blood bound to albumin (Fig. 30.1). In cells, they are converted to fatty acyl coenzyme A (fatty acyl CoA) derivatives by acyl CoA synthetases. The activated acyl group is transported into the mitochondrial matrix bound to carnitine, where fatty acyl CoA is regenerated. In the pathway of β-oxidation, the fatty acyl group is sequentially oxidized to yield FAD(2H), NADH, and acetyl CoA. Subsequent oxidation of NADH and FAD(2H) in the electron transport chain, and oxidation of acetyl CoA to CO2 in the tricarboxylic acid (TCA) cycle, generates ATP from oxidative phosphorylation.
FIGURE 30.1 Overview of mitochondrial long-chain fatty acid metabolism. (1) Fatty acid–binding proteins (FaBPs) transport fatty acids across the plasma membrane and bind them in the cytosol. (2) Fatty acyl coenzyme A (Fatty acyl CoA) synthetase activates fatty acids to fatty acyl CoAs. (3) Carnitine transports the activated fatty acyl group into mitochondria. (4) β-Oxidation generates NADH, FAD(2H), and acetyl coenzyme A (acetyl CoA). (5) In the liver, acetyl CoA can be converted to ketone bodies. ATP, adenosine triphosphate; FAD(2H), reduced flavin adenine dinucleotide; GTP, guanosine triphosphate; NADH, nicotinamide adenine dinucleotide; TCA, tricarboxylic acid.
Many fatty acids have structures that require variations of this basic pattern. Long-chain fatty acids that are unsaturated fatty acids generally require additional isomerization and oxidation–reduction reactions to rearrange their double bonds during β-oxidation. Metabolism of water-soluble medium-chain-length fatty acids does not require carnitine and occurs only in the liver. Odd-chain-length fatty acids undergo β-oxidation to the terminal three-carbon propionyl CoA, which enters the TCA cycle as succinyl CoA.
Fatty acids that do not readily undergo mitochondrial β-oxidation are oxidized first by alternate routes that convert them to more suitable substrates or to urinary excretion products. Excess fatty acids may undergo microsomal ω-oxidation, which converts them to dicarboxylic acids that appear in urine. Very-long-chain fatty acids (both straight-chain and branched fatty acids such as phytanic acid) are whittled down to size in peroxisomes. Peroxisomal α- and β-oxidation generates hydrogen peroxide (H2O2), NADH, acetyl CoA, or propionyl CoA and a short- to medium-chain-length acyl CoA. The acyl CoA products are transferred to mitochondria to complete their metabolism.
In the liver, much of the acetyl CoA generated from fatty acid oxidation is converted to the ketone bodies, acetoacetate and β-hydroxybutyrate, which enter the blood (see Fig. 30.1). In other tissues, these ketone bodies are converted to acetyl CoA, which is oxidized in the TCA cycle. The liver synthesizes ketone bodies but cannot use them as a fuel.
The rate of fatty acid oxidation is linked to the rate of NADH, FAD(2H), and acetyl CoA oxidation and thus to the rate of oxidative phosphorylation and ATP utilization. Additional regulation occurs through malonyl CoA, which inhibits formation of the fatty acyl carnitine derivatives. Fatty acids and ketone bodies are used as fuel when their level increases in the blood, which is determined by hormonal regulation of adipose tissue lipolysis.
THE WAITING ROOM 
Otto S. was disappointed that he did not place in his 5-km race and has decided that short-distance running is probably not right for him. After careful consideration, he decides to train for the marathon by running 12 miles three times per week. He is now 13 lb over his ideal weight, and he plans on losing this weight while studying for his Pharmacology finals. He considers a variety of dietary supplements to increase his endurance and selects one that contains carnitine, CoQ, pantothenate, riboflavin, and creatine.
Lola B. is a 16-year-old girl. Since age 14 months, she has experienced recurrent episodes of profound fatigue associated with vomiting and increased perspiration, which required hospitalization. These episodes occurred only if she fasted for more than 8 hours. Because her mother gave her food late at night and woke her early in the morning for breakfast, Lola B.’s physical and mental development had progressed normally.
On the day of admission for this episode, Lola B. had missed breakfast, and by noon, she was extremely fatigued, nauseated, and weak. She was unable to hold any food in her stomach and was rushed to the hospital, where an infusion of glucose was started intravenously. Her symptoms responded dramatically to this therapy.
Dianne A., a 27-year-old woman with type 1 diabetes mellitus, had been admitted to the hospital in a ketoacidotic coma a year ago (see Chapter 4). She had been feeling drowsy and had been vomiting for 24 hours before that admission. At the time of admission, she was clinically dehydrated, her blood pressure was low, and her breathing was deep and rapid (Kussmaul breathing). Her pulse was rapid, and her breath had the odor of acetone. Her arterial blood pH was 7.08 (reference range, 7.36 to 7.44), and her blood ketone body levels were 15 mM (normal is approximately 0.2 mM for a person on a normal diet).
I. Fatty Acids as Fuels
The fatty acids oxidized as fuels are principally long-chain fatty acids released from adipose tissue triacylglycerol stores between meals, during overnight fasting, and during periods of increased fuel demand (e.g., during exercise). Adipose tissue triacylglycerols are derived from two sources: dietary lipids and triacylglycerols synthesized in the liver. The major fatty acids oxidized are the long-chain fatty acids, palmitate, oleate, and stearate because they are highest in dietary lipids and are also synthesized in the human.
Between meals, a decreased insulin level and increased levels of insulin counterregulatory hormones (e.g., glucagon) activate lipolysis and free fatty acids are transported to tissues bound to serum albumin. Within tissues, energy is derived from oxidation of fatty acids to acetyl CoA in the pathway of β-oxidation. Most of the enzymes involved in fatty acid oxidation are present as two or three isoenzymes, which have different but overlapping specificities for the chain length of the fatty acid. Metabolism of unsaturated fatty acids, odd-chain-length fatty acids, and medium-chain-length fatty acids requires variations of this basic pattern. The acetyl CoA produced from fatty acid oxidation is principally oxidized in the TCA cycle or converted to ketone bodies in the liver.
A. Characteristics of Fatty Acids Used as Fuels
Fat constitutes approximately 38% of the calories in the average North American diet. Of this, more than 95% of the calories are present as triacylglycerols (three fatty acids esterified to a glycerol backbone). During ingestion and absorption, dietary triacylglycerols are broken down into their constituents and then reassembled for transport to adipose tissue in chylomicrons (see Chapter 29). Thus, the fatty acid composition of adipose triacylglycerols varies with the type of food consumed.
The most common dietary fatty acids are the saturated long-chain fatty acids palmitate (C16) and stearate (C18), the monounsaturated fatty acid oleate (C18:1), and the polyunsaturated essential fatty acid linoleate (C18:2) (to review fatty acid nomenclature, consult Chapter 5). Animal fat contains principally saturated and monounsaturated long-chain fatty acids, whereas vegetable oils contain linoleate and some longer-chain and polyunsaturated fatty acids. They also contain smaller amounts of branched-chain and odd-chain-length fatty acids. Medium-chain-length fatty acids are present principally in dairy fat (e.g., milk and butter), maternal milk, and vegetable oils.
Adipose tissue triacylglycerols also contain fatty acids synthesized in the liver, principally from excess calories ingested as glucose. The pathway of fatty acid synthesis generates palmitate, which can be elongated to form stearate or unsaturated to form oleate. These fatty acids are assembled into triacylglycerols and transported to adipose tissue as the lipoprotein VLDL (very-low-density lipoprotein).
B. Transport and Activation of Long-Chain Fatty Acids
Long-chain fatty acids are hydrophobic and, therefore, water insoluble. In addition, they are toxic to cells because they can disrupt the hydrophobic bonding between amino acid side chains in proteins. Consequently, they are transported in the blood and in cells bound to proteins.
1. Cellular Uptake of Long-Chain Fatty Acids
During fasting and other conditions of metabolic need, long-chain fatty acids are released from adipose tissue triacylglycerols by lipases. They travel in the blood bound in the hydrophobic binding pocket of albumin, the major serum protein (see Fig. 30.1).
Fatty acids enter cells both by a saturable transport process and by diffusion through the lipid plasma membrane. A fatty acid–binding protein in the plasma membrane facilitates transport. An additional fatty acid–binding protein binds the fatty acid intracellularly and may facilitate its transport to the mitochondrion. The free fatty acid concentration in cells is, therefore, extremely low.
2. Activation of Long-Chain Fatty Acids
Fatty acids must be activated to acyl CoA derivatives before they can participate in β-oxidation and other metabolic pathways (Fig. 30.2). The process of activation involves an acyl CoA synthetase (also called a thiokinase) that uses ATP energy to form the fatty acyl CoA thioester bond. In this reaction, the β-bond of ATP is cleaved to form a fatty acyl adenosine monophosphate (AMP) intermediate and pyrophosphate (PPi). Subsequent cleavage of PPi helps to drive the reaction.
FIGURE 30.2 Activation of a fatty acid by a fatty acyl coenzyme A (fatty acyl CoA) synthetase. The fatty acid is activated by reacting with adenosine triphosphate (ATP) to form a high-energy fatty acyl adenosine monophosphate (AMP) and pyrophosphate. The AMP is then exchanged for coenzyme A (CoA). Pyrophosphate is cleaved by a pyrophosphatase. Pi, inorganic phosphate.
The acyl CoA synthetase that activates long-chain fatty acids, 12 to 20 carbons in length, is present in three locations in the cell: the endoplasmic reticulum, outer mitochondrial membranes, and peroxisomal membranes (Table 30.1). This enzyme has no activity toward C22 or longer fatty acids and little activity below C12. In contrast, the synthetase for activation of very-long-chain fatty acids is present in peroxisomes, and the medium-chain-length fatty acid–activating enzyme is present only in the mitochondrial matrix of liver and kidney cells.
TABLE 30.1 Chain-Length Specificity of Fatty Acid Activation and Oxidation Enzymes
ENZYME | CHAIN LENGTH | COMMENTS |
Acyl CoA synthetases | ||
Very long chain | 14–26 | Found only in peroxisomes. |
Long chain | 12–20 | Enzymes present in membranes of endoplasmic reticulum, mitochondria, and peroxisomes to facilitate different metabolic routes of acyl CoAs. |
Medium chain | 6–12 | Exists as many variants, present only in mitochondrial matrix of kidney and liver. Also involved in xenobiotic metabolism. |
Acetyl | 2–4 | Present in cytoplasm and possibly mitochondrial matrix. |
Acyltransferases | ||
CPTI | 12–16 | Although maximum activity is for fatty acids 12–16 carbons long, it also acts on many smaller acyl CoA derivatives. |
Medium chain (octanoylcarnitine transferase) | 6–12 | Substrate is medium-chain acyl CoA derivatives generated during peroxisomal oxidation. |
Carnitine acetyltransferase | 2 | High level in skeletal muscle and heart to facilitate use of acetate as a fuel. |
Acyl CoA dehydrogenases | ||
VLCAD | 14–20 | Present in inner mitochondrial membrane. |
LCAD | 12–18 | Members of same enzyme family, which also includes acyl CoA dehydrogenases for carbon skeleton of branched-chain amino acids. Low expression of LCAD in humans; VLCAD is the predominant acyl CoA dehydrogenase for long-chain fatty acids. |
MCAD | 4–12 | |
SCAD | 4–6 | |
Other enzymes | ||
Enoyl CoA hydratase, short chain | >4 | Also called crotonase. Activity decreases with increasing chain length. |
L-3-Hydroxyacyl CoA dehydrogenase, short chain | 4–16 | Activity decreases with increasing chain length. |
Acetoacetyl CoA thiolase | 4 | Specific for acetoacetyl CoA. |
Mitochondrial trifunctional protein | 12–16 | Complex of long-chain enoyl hydratase, L-3-hydroxyacyl CoA dehydrogenase, and a thiolase with broad specificity. Most active with longer chains. |
CPTI, carnitine palmitoyltransferase 1; LCAD, long-chain acyl CoA dehydrogenase; MCAD, medium-chain acyl CoA dehydrogenase; SCAD, short-chain acyl CoA dehydrogenase; VLCAD, very-long-chain acyl CoA dehydrogenase.
3. Fates of Fatty Acyl Coenzyme A
Fatty acyl CoA formation, like the phosphorylation of glucose, is a prerequisite to metabolism of the fatty acid in the cell (Fig. 30.3). The multiple locations of the long-chain acyl CoA synthetase reflect the location of different metabolic routes taken by fatty acyl CoA derivatives in the cell (e.g., triacylglycerol and phospholipid synthesis in the endoplasmic reticulum, oxidation and plasmalogen synthesis in the peroxisome, and β-oxidation in mitochondria). In the liver and some other tissues, fatty acids that are not being used for energy generation are reincorporated (re-esterified) into triacylglycerols.
FIGURE 30.3 Major metabolic routes for long-chain fatty acyl coenzyme As (fatty acyl CoAs). Fatty acids are activated to acyl CoA compounds for degradation in mitochondrial β-oxidation, or incorporation into triacylglycerols or membrane lipids. When β-oxidation is blocked through an inherited enzyme deficiency, or metabolic regulation, excess fatty acids are diverted into triacylglycerol synthesis.
4. Transport of Long-Chain Fatty Acids into Mitochondria
Carnitine serves as the carrier that transports activated long-chain fatty acyl groups across the inner mitochondrial membrane (Fig. 30.4). Carnitine acyltransferases are able to reversibly transfer an activated fatty acyl group from CoA to the hydroxyl group of carnitine to form an acylcarnitine ester. The reaction is reversible, so the fatty acyl CoA derivative can be regenerated from the carnitine ester.
FIGURE 30.4 Structure of fatty acylcarnitine. Carnitine palmitoyltransferases catalyze the reversible transfer of a long-chain fatty acyl group from the fatty acyl coenzyme A (fatty acyl CoA) to the hydroxyl group of carnitine. The atoms in the green box originate from the fatty acyl CoA.
Carnitine palmitoyltransferase I (CPTI; also called carnitine acyltransferase I, CATI), the enzyme that transfers long-chain fatty acyl groups from CoA to carnitine, is located on the outer mitochondrial membrane (Fig. 30.5). Fatty acylcarnitine crosses the inner mitochondrial membrane with the aid of a translocase. The fatty acyl group is transferred back to CoA by a second enzyme, carnitine palmitoyltransferase II (CPTII or CATII). The carnitine released in this reaction returns to the cytosolic side of the mitochondrial membrane by the same translocase that brings fatty acylcarnitine to the matrix side. Long-chain fatty acyl CoA, now located within the mitochondrial matrix, is a substrate for β-oxidation.
FIGURE 30.5 Transport of long-chain fatty acids into mitochondria. The fatty acyl coenzyme A (fatty acyl CoA) crosses the outer mitochondrial membrane. Carnitine palmitoyltransferase I (CPTI) in the outer mitochondrial membrane transfers the fatty acyl group to carnitine and releases CoASH. The fatty acyl carnitine is translocated into the mitochondrial matrix as carnitine moves out. Carnitine palmitoyltransferase II on the inner mitochondrial membrane transfers the fatty acyl group back to CoASH, to form fatty acyl CoA in the matrix. AMP, adenosine monophosphate; ATP, adenosine triphosphate; CoA, coenzyme A; PPi, pyrophosphate.
Carnitine is obtained from the diet or synthesized from the side chain of lysine by a pathway that begins in skeletal muscle and is completed in the liver. The reactions use S-adenosylmethionine to donate methyl groups, and vitamin C (ascorbic acid) is also required for these reactions. Skeletal muscles have a high-affinity uptake system for carnitine, and most of the carnitine in the body is stored in skeletal muscle.
C. 𝛃-Oxidation of Long-Chain Fatty Acids
The oxidation of fatty acids to acetyl CoA in the β-oxidation spiral conserves energy as FAD(2H) and NADH. FAD(2H) and NADH are oxidized in the electron transport chain, generating ATP from oxidative phosphorylation. Acetyl CoA is oxidized in the TCA cycle or converted to ketone bodies.
1. The β -Oxidation Spiral
The fatty acid β-oxidation pathway sequentially cleaves the fatty acyl group into two-carbon acetyl CoA units, beginning with the carboxyl end attached to CoA (Fig. 30.6). Before cleavage, the β-carbon is oxidized to a keto group in two reactions that generate NADH and FAD(2H); thus, the pathway is called β-oxidation. As each acetyl group is released, the cycle of β-oxidation and cleavage begins again, but each time, the fatty acyl group is two carbons shorter.
FIGURE 30.6 Overview of β-oxidation. Oxidation at the β-carbon is followed by cleavage of the α–β bond, releasing acetyl coenzyme A (acetyl CoA) and a fatty acyl coenzyme A (fatty acyl CoA) that is two carbons shorter than the original. The carbons cleaved to form acetyl CoA are shown in red. Successive spirals of β-oxidation completely cleave an even-chain fatty acyl CoA to acetyl CoA.
The β-oxidation pathway consists of four separate steps or reactions (Fig. 30.7).
FIGURE 30.7 Steps of β-oxidation. The four steps are repeated until an even-chain fatty acid is completely converted to acetyl coenzyme A (acetyl CoA). The flavin adenine dinucleotide (FAD[2H]) and nicotinamide adenine dinucleotide (NADH) are reoxidized by the electron transport chain, producing adenosine triphosphate (ATP).
- In the first step, a double bond is formed between the β- and α-carbons by an acyl CoA dehydrogenase that transfers electrons to FAD. The double bond is in the trans configuration (a ∆2–trans double bond).
- In the next step, an –OH from water is added to the β-carbon, and an –H from water is added to the α-carbon. The enzyme is called an enoyl CoA hydratase (hydratases add the elements of water, and “-ene” in a name denotes a double bond).
- In the third step of β-oxidation, the hydroxyl group on the β-carbon is oxidized to a ketone by a hydroxyacyl CoA dehydrogenase. In this reaction, as in the conversion of most alcohols to ketones, the electrons are transferred to NAD+ to form NADH.
- In the last reaction of the sequence, the bond between the β- and α-carbons is cleaved by a reaction that attaches CoASH to the β-carbon, and acetyl CoA is released. This is a thiolytic reaction (“lysis” refers to breakage of the bond, and “thio” refers to the sulfur), catalyzed by enzymes called β-ketothiolases. The release of two carbons from the carboxyl end of the original fatty acyl CoA produces acetyl CoA and a fatty acyl CoA that is two carbons shorter than the original. It is of interest to note that the β-oxidation spiral uses the same reaction types seen in the TCA cycle in the conversion of succinate to oxaloacetate.
- The enzyme activities that catalyze the last three steps of β-oxidation are all part of one protein complex, known as the mitochondrial trifunctional protein (TFP). The complex consists of eight proteins, four each of an α-subunit (encoded by the HADHA gene) and four each of a β-subunit (encoded by the HADHB gene). The MTP complex is associated with the inner mitochondrial membrane.
- In the third step of β-oxidation, the hydroxyl group on the β-carbon is oxidized to a ketone by a hydroxyacyl CoA dehydrogenase. In this reaction, as in the conversion of most alcohols to ketones, the electrons are transferred to NAD+ to form NADH.
The shortened fatty acyl CoA repeats these four steps until all of its carbons are converted to acetyl CoA. β-Oxidation is thus a spiral rather than a cycle. In the last spiral, cleavage of the four-carbon fatty acyl CoA (butyryl CoA) produces two molecules of acetyl CoA. Thus, an even-chain fatty acid such as palmitoyl CoA, which has 16 carbons, is cleaved seven times, producing 7 FAD(2H), 7 NADH, and 8 molecules of acetyl CoA.
2. Energy Yield of β -Oxidation
Like the FAD in all flavoproteins, FAD(2H) bound to the acyl CoA dehydrogenases is oxidized back to FAD without dissociating from the protein (Fig. 30.8). Electron transfer flavoproteins (ETFs) in the mitochondrial matrix accept electrons from the enzyme-bound FAD(2H) and transfer these electrons to ETF-QO (electron transfer flavoprotein–CoQ oxidoreductase) in the inner mitochondrial membrane. ETF-QO, also a flavoprotein, transfers the electrons to CoQ in the electron transport chain. Oxidative phosphorylation thus generates approximately 1.5 ATP for each FAD(2H) produced in the β-oxidation spiral.
FIGURE 30.8 Transfer of electrons from acyl coenzyme A (acyl CoA) dehydrogenase to the electron transport chain. A flavin adenine dinucleotide (FAD) is tightly bound to each protein in these three electron transfer reactions. ETF, electron transfer flavoprotein; ETF-QO, electron transferring flavoprotein–coenzyme Q oxidoreductase.
The total energy yield from the oxidation of 1 mol of palmitoyl CoA to 8 mol of acetyl CoA is, therefore, 28 mol of ATP: 1.5 for each of the 7 FAD(2H), and 2.5 for each of the 7 NADH. To calculate the energy yield from oxidation of 1 mol of palmitate, two ATP need to be subtracted from the total because two high-energy phosphate bonds are cleaved when palmitate is activated to palmitoyl CoA.
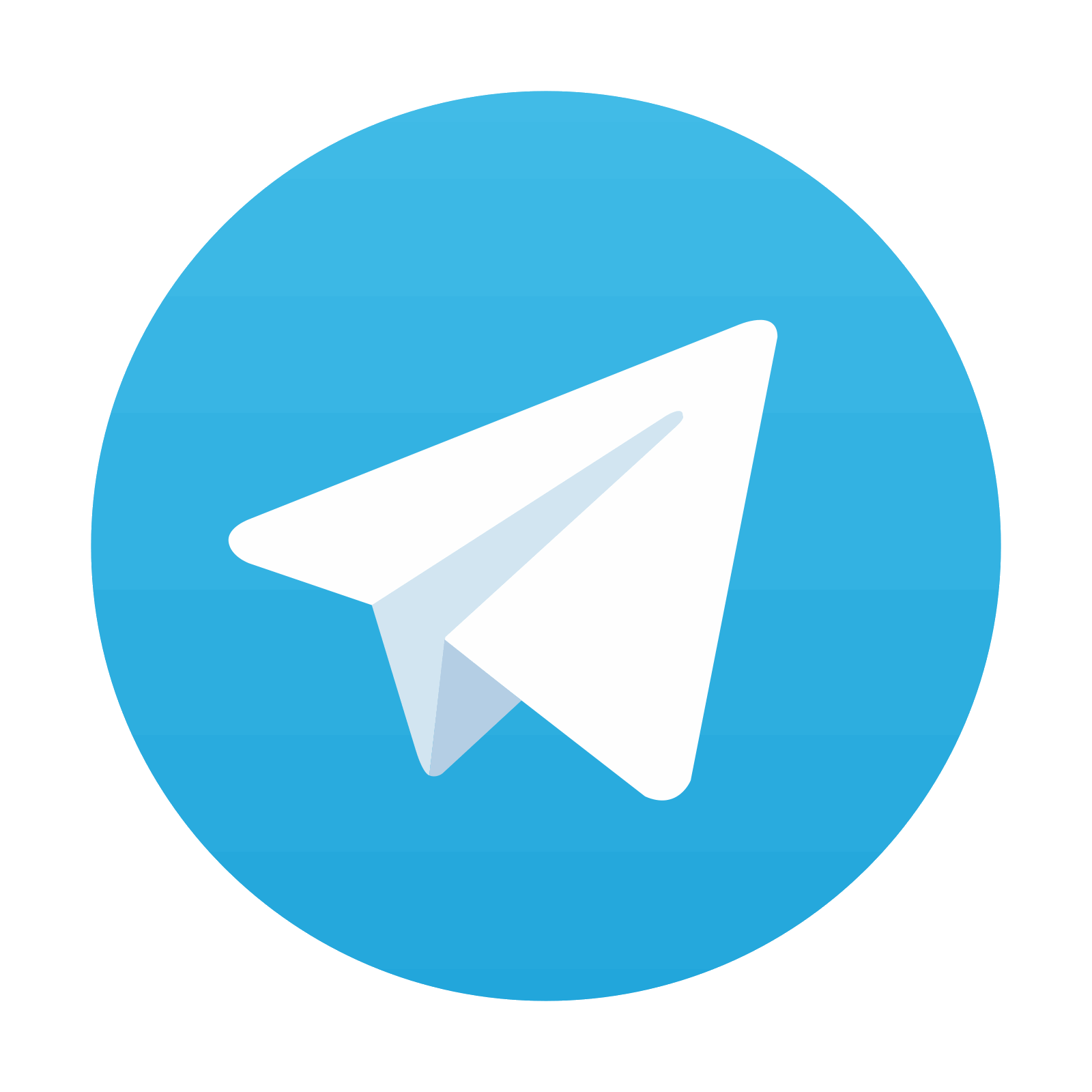
Stay updated, free articles. Join our Telegram channel
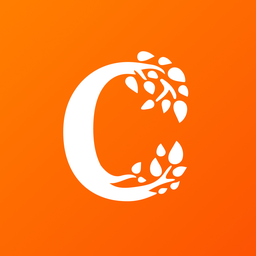
Full access? Get Clinical Tree
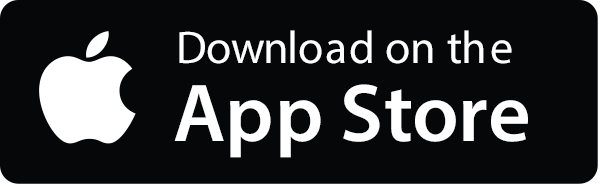
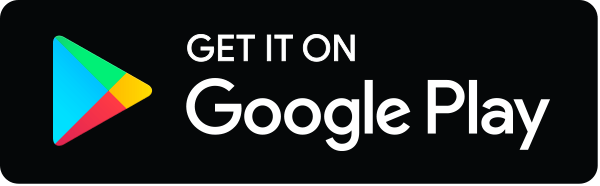